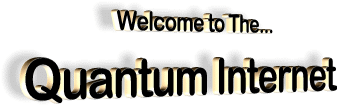
by Davide Castelvecchi
August 16, 2008
Vol.174 #4
from
ScienceNews Website
Spanish version
Quantum Encryption is
Here,
But The Laws of Physics Can Do
Much More
Than Protect Privacy
A stylish new way of surfing the
Internet is coming to Vienna this fall.
Researchers plan to flip the switch on
the next step toward a quantum version of the Internet. They will
build a network allowing users to send each other messages as
virtually unbreakable ciphers, with privacy protected by the laws of
quantum physics.
The Vienna net is admittedly just a prototype for research purposes.
It is also not yet a true
quantum version of the Internet. Although
it can transmit ordinary data with quantum security, it can’t
transfer quantum information, which encodes the states of objects
that obey quantum rules.
Such a breakthrough might be years off,
but it’s getting closer.
Truth be told, it’s not completely clear what a fully quantum
Internet would be good for. In fact, at first it even sounds like a
really bad idea. Quantum information is notoriously wobbly. An
object tends to live in a superposition of states - for example, an
electron can spin in two directions at once, or an atom can be
simultaneously in two different places - until interaction with the
rest of the world forces the object to pick one state.
Quantum reality is a limbo of coexisting
possibilities.
And because any measurement done of a quantum system changes the
system’s state irreversibly, quantum information is different every
time it’s read. That makes it impossible, for example, to copy,
broadcast or back up quantum data.
But the eccentric physics could also impart unique strengths to
networks.
While each data bit in an ordinary computer takes the
value 0 or 1, the units of quantum information, called
quantum bits,
or qubits for short, can take both values simultaneously. A quantum
Internet could transfer software and data between future (and
futuristic) quantum computers, which could outperform ordinary
computers by running multiple operations at once, in superposition.
And the network could lead to new kinds
of social interactions - such as letting
quantum physics pick a
presidential candidate who pleases the most voters or allowing
people to donate to a cause based on whether others donate as well -
and do so with absolute secrecy.
Perhaps - and this inches toward Star Trek territory - some day a
quantum net could even “beam up” a physical object. All the
information needed to re-create the object, such as its shape and
energy, would be transferred elsewhere, leaving just chaos behind.
In the meantime, when the switch is flipped October 8, the Vienna
net will demonstrate how quantum physics can keep ordinary
information, such as an e-mail or the balance of a checking account,
safe from prying eyes.
This latest step toward the quantum Internet is a limited network
backbone that will often run at the speed of a 1980s modem. To plug
into it, a user would need to buy expensive gear and link an optical
fiber to one of the backbone’s five nodes. But it’s a step.
Meanwhile, most of the basic technical ingredients of a truly
quantum Internet have now been demonstrated, at least in the lab.
In
particular, researchers have created various types of “quantum
memory,” in which light pulses traveling through an optical fiber
essentially slow to a halt, a crucial requirement for the quantum
version of an Internet router.
So it may be just a matter of time
before scientists can start beaming up stuff - or at least data.
“I’m optimistic that within a few
years we’ll be able to build at least a lab demonstration of a
quantum network,” says Mikhail Lukin of Harvard University.
A solid
quantum key
In tunnels stretching under Vienna and the Danube river, pulses of
light will be beamed this October along tens of kilometers of
existing optical fibers owned by German engineering conglomerate
Siemens.
A collaboration of more than 40
universities, companies and research institutions will piece
together technologies to link five Siemens buildings, four of them
scattered across the city and one 85 kilometers away in the town of
St. Pölten.
The building-to-building connections will use a number of quantum
encryption systems to pass the information, many of them inspired by
a version of quantum encryption first proposed in 1991 by
Artur Ekert, now at the National University of Singapore.
With Ekert’s procedure, the sender and
the receiver, conventionally called Alice and Bob, use both a
quantum connection and a classical one, which could be the good-old
Internet or a phone line.
Through the quantum connection, Alice and Bob establish a common
encryption key - a secret sequence of data bits that Alice will use
to scramble her message, and Bob to unscramble it.
Alice can then
send her scrambled message to Bob through the classical connection,
for example as an e-mail attachment.
To someone who doesn’t know the key, Alice’s message would look like
a random sequence of bits. Even the most sophisticated computer
imaginable wouldn’t be able to crack it. But Bob knows the key, so
he can unscramble the message.
Keeping the key secret as they create it is the crucial part, and
here’s where Ekert exploits quantum physics - specifically, a weird
phenomenon called
quantum entanglement. In quantum physics, each of
two objects can exist in its own state, or the objects’ states can
be entangled, meaning that, while separate, they are not independent
of each other.
Take photons, the elementary particles that form electromagnetic
radiation, including light.
Photons wiggle sideways as they zip
along an optical fiber. Two photons can wiggle in independent
directions, called linear polarizations. But two photons can also be
entangled, so that, for example, when one photon is polarized
vertically, the other must be polarized horizontally, and vice
versa.
In Ekert-style encryption, a laser device creates pairs of entangled
photons and sends (along the fiber-optic cable) one photon from each
entangled pair to Alice and the other one to Bob.
Because photons in each pair have correlated polarizations, Alice
and Bob could now turn that information into a common key, which for
example could contain a 0 for each vertically polarized photon and a
1 for each horizontally polarized one.
However, Alice and Bob also want to be sure the photons they are
using haven’t been intercepted by an eavesdropper, inevitably
referred to as Eve. Any Eve who intercepts the photons, trying to
steal the key, will change the photons’ states, or even destroy
them, since it’s impossible to measure the state of a quantum system
without changing it irreversibly.
Alice and Bob, over the phone, will then
compare notes on their test photons. If they notice discrepancies,
they’ll know Eve was there, so they’ll throw away the key and start
again.
Quantum encryption systems are now available commercially (i.e.
Pretty Good Privacy -
PGP). Some are
owned by banking institutions, for example, and one was used last
fall in Switzerland to transmit electoral data from an electronic
polling station.
So far, though, these links have mostly
been point-to-point rather than networks with multiple users.
With a network of quantum-encrypted lines such as the one being
built in Vienna, users will just need to link to the node closest to
them. When one user wants to send a secret message to another, the
message will travel in encrypted form from the first user to an
entry node. There, the message will be decrypted and then encrypted
again (using a new key) to be sent to the next node.
The same will happen at every node in
between, until the message reaches its destination.
Privacy will be guaranteed, as long as the locations of the sender,
the receiver and the intermediate nodes stay protected from
intrusion. (By routing messages through multiple nodes
simultaneously and using some mathematical tricks, the network will
actually guarantee privacy even if one of the nodes is broken into.)
This piecemeal encryption - a solution also adopted on a smaller
scale in a Boston-area quantum network laid out in 2003 - is needed
because of a fundamental limitation with transmitting photons.
Quantum RAM
Sharing an encryption key between any two users requires sending
single photons - entangled photons in the case of Ekert’s scheme.
But something as small as a photon
easily gets lost or absorbed even in the highest-quality optical
fiber, says Norbert Lütkenhaus of the University of Waterloo
in Canada, a physicist who helped design Vienna’s quantum net.
“You lose one-half of the photons
every 15 kilometers,” he says.
Establishing a key thus becomes
exponentially slower as the distance increases. Lütkenhaus
calculates that 25 kilometers is still a good distance for decently
efficient quantum communication, but beyond that distance a
different solution is needed.
In the case of ordinary optical communications, the problem of
photon loss is easily solved by adding “repeaters” along the line -
gadgets that receive weakened laser pulses and replace them with
stronger ones.
But ordinary repeaters don’t work for quantum systems
such as single photons.
For one thing, as Lukin points out,
“If you sent a single photon, if
it’s lost, there’s nothing left to amplify.”
And if the photon does arrive at the
node, the laws of quantum physics forbid fully copying its quantum
state, so some of the photon’s information will inevitably be lost.
In particular, if the photon was
entangled with another photon somewhere else, the entanglement will
be lost.
However, in 2001 Lukin and his collaborators envisioned a way to get
around this problem by creating entangled pairs from photons that
are far apart. If realized, their scheme would enable long-distance,
quantum-encrypted communication.
If photons can be entangled over long distances, they could enable
people to interact in ways that just aren’t possible within the
realm of classical physics.
One power of entanglement is that it makes quantum
teleportation
possible. That’s a nearly magical way of transferring the quantum
state of an object onto another object, possibly far away. Say Alice
has a photon X, which she wants to teleport to Bob.
Alice also has a photon Y, which is
entangled with a photon Z owned by Bob. Alice then makes her two
photons interact. That way, the state of X becomes entangled with
the state of Y, and thus with the state of Z.
Alice then destroys X and Y by measuring their states, and she calls
up Bob to tell him the results. Using that information, Bob can now
twist the state of Z to make it identical to the original state of
X. Alice has sacrificed the two photons in her possession, but as a
result, Bob now has an exact copy of the original photon, photon X.
Lukin’s idea to create long-distance entanglement relies on yet
another trick called entanglement swapping. In entanglement
swapping, each of two sources produces a pair of entangled photons.
The photons from the first source, say A
and B, are not entangled with those from the second source, say C
and D. Next, B and C are brought to the same detector. There, B and
C interact and are destroyed, causing A and D to become entangled
even though they were never close to each other.
Repeated applications of entanglement swapping over a chain of nodes
can create pairs of entangled photons that are farther and farther
from each other. Eventually, all photons are destroyed, except for
the ones at the opposite ends of the chain. Those two end up
entangled.
The method seems fail-safe on paper, but in practice, at each step
at least some of the photons have a high chance of getting lost. But
if one could somehow store pairs of photons that have successfully
been entangled while other pairs are still being generated,
long-distance entanglement would become possible at a reasonable
speed.
The key to quantum networking, then, is
the ability to keep entangled photons in a sort of quantum RAM.
Catch and
release
In 2001, Lukin and his collaborators, and an independent Harvard
group led by Lene Hau, created the first rudimentary quantum
memory, essentially by slowing light to a crawl inside clouds of
atoms (SN: 1/27/01, p. 52).
Since then, several groups have
performed ever-more-advanced quantum-memory tricks.
For example, groups led by Lukin,
Alex Kuzmich of the Georgia Institute of Technology in Atlanta
and Jeff Kimble of the California Institute of Technology in
Pasadena were able to take a photon emitted by one atom cloud and
store it in another atom cloud.
And last September, Christopher
Monroe and his team at the University of Maryland in College
Park were able to entangle two
qubits made of single ions.
Most recently, in the March 6 Nature, a team led by Kimble described
what may be the most advanced kind of quantum memory to date. The
researchers captured two entangled photon states in atom clouds and
were able to release the states on demand.
The photon states remained entangled
during the capture and release.
“We put entanglement into matter and
then read it out,” says Kimble’s coauthor Julien Laurat, who was
then a colleague of Kimble’s at Caltech but is now at the Pierre
and Marie Curie University in Paris.
First, Kimble, Laurat and their
colleagues shot photons one at a time at a semitransparent mirror.
In this situation each photon, presented with the choice of bouncing
off or zipping through, will not make up its mind right away.
Instead, it will split its path into two, a superposition of both
possibilities. Only when forced to interact, for example by running
into a detector, will the photon appear all in one place or in the
other. Because these two measurements are mutually exclusive rather
than independent, the two paths are entangled states.
Next, the researchers trapped each of the virtual photons in a cloud
of cesium atoms. Using a laser pulse, the physicists turned the
clouds transparent, to allow the photons in. When the physicists
turned the laser off, the clouds went back to opaque, trapping the
photons inside.
That forced the photons to virtually come to a stop,
as their quantum states became enmeshed with the quantum states of
the clouds.
So the clouds themselves became entangled.
The team was able to store the quantum information - preserve the
entanglement - for up to 10 microseconds. A second laser pulse made
the gas transparent again, allowing the two virtual photons to
escape and continue on their paths. The physicists were able to
check that the two photon states were still entangled.
What’s missing now, Laurat says, is the ability to entangle two
separate qubits by entanglement swapping.
Still, Lukin says, the
Caltech result was “an important step.”
In another recent result, Kuzmich and his collaborators induced a
cloud of atoms to emit two photons at once, with wavelengths that
were each optimized for different tasks - for transmission through
an optical fiber and for storage in another qubit. Typically, single
photons emitted by atom clouds tend to have wavelengths too short
for efficient telecommunications, Kuzmich says.
According to Lukin, eventually, a practical quantum memory will need
to store information on some kind of solid support. In this respect,
he says, single-atom impurities in artificial diamond are one of the
most promising candidates, since they would require no sophisticated
laboratory to handle (SN: 4/5/08, p. 216).
Most of the pieces needed to put together a quantum Internet now
exist, and the challenge will be to make them work together
efficiently.
With the best technology available so far, a working
prototype might end up costing as much as $100 million, and might be
able to send just one qubit per minute, Kuzmich says.
A more sensible question might be: What would a quantum Internet be
good for? So far, the main motivation for researchers has been to
provide secure communications.
But a quantum Internet might some day do
things that, until recently, would have sounded like complete
science fiction.
Citations
-
Poppe, A., M. Peev, and O.
Maurhart 2008. Outline of the SECOQC
quantum-key-distribution network in Vienna.
International Journal of Quantum Information
6(April):209. Available at
[Go to].
-
Bradley, A.S., et al. 2007.
Teleportation of massive particles without shared
entanglement. Available at
[Go to].
-
Chanelière, T., D.N...
and A. Kuzmich 2007. Quantum interference of
electromagnetic fields from remote quantum memories.
Physical Review Letters 98(March 16):113602. Available
at
[Go to].
-
Moehring, D.L... and C.
Monroe 2007. Entanglement of single-atom quantum bits at
a distance. Nature 446(Sept. 6):68.
doi:10.1038/nature06118
-
Duan, L. M., M.D. Lukin, et
al. 2001. Long-distance quantum communication with
atomic ensembles and linear optics. Nature 414(Nov.
22):413. Available at
[Go to].
doi:10.1038/35106500
-
Choi, K.S... J. Laurat, and H.J. Kimble 2008. Mapping photonic
entanglement into and out of a quantum memory. Nature
452(March 6):67. doi:10.1038/nature06670
[Go to]
|