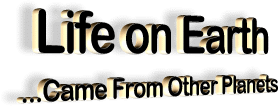
by Rhawn Joseph, Ph.D.
Emeritus, Brain Research
Laboratory, Northern California
2009
Journal of Cosmology, 2009, Vol 1, pages 1-56
Cosmology 2009
Peer Reviewed - With Open Peer Commentary
from
JournalOfCosmology Website
ABSTRACT
A comprehensive theory based on a review of scientific findings
published in prestigious scientific journals, is presented to
explain how life on Earth came from other planets.
Life appeared a few hundred million
years after the Earth's creation during a period of heavy
bombardment.
Life on Mars may have appeared near the same time.
Microbes are adapted for surviving the hazards of space, including
ejection from and landing upon a planet. Microbial fossils have been
discovered in fifteen carbonaceous
chondrites, most impacted by
supernova.
The Sun and Earth were created from a
nebular cloud and protoplanetary disc, the remnants of an exploding
star and its planets which may have harbored life. When the parent
star became a red giant, its solar winds blew away planetary
atmospheres along with airborne microbes, which were deposited in a
growing nebular cloud.
Because the red giant lost 40% to 80% of
its mass and its gravitational influences were reduced, its planets
increased orbital distances or were ejected prior to supernova and
may not have been atomized. The inner layers of a nebular cloud and
protoplanetary disk protects against radiation and extreme cold
enabling spores to survive. Microbes may have also survived within
planetary debris which bombarded the Earth.
As only life can produce life, then life
on Earth also came from life which may have originated on planets
which orbited the parent star.
1.
Introduction
"Very tiny animals result from
the corruption of mortal things, arise from defects of dead
bodies, or from excrements, or from putrefaction of dead
bodies."
-St. Augustine, Catholic
Bishop, Church Father, Catholic Saint.
The origin of life is unknown, though
speculation abounds.
For thousands of years philosophers,
scientists, and theologians have argued that Earthly life comes from
non-life. This belief has been part of Catholic Church dogma since
the 4th century (Augustine, 1957).
Although known by many names (e.g.
vitalism, spontaneous generation, the organic soup, abiogenesis),
claims as to the abiogenic origins of Earthly life are based on
belief in super natural forces and are firmly rooted in the
Jewish-Christian Bible, Genesis, Chapter 1:
"And God said, Let the earth bring
forth the living creature after his kind, cattle, and creeping
thing, and beast of the earth after his kind: and it was so."
Thus, according to the early Church
Fathers, god gave the Earth special life giving powers for
spontaneously generating plants and animals:
"The earth is said then to have
produced grass and trees causally, that is, to have received the
power of producing".
(Augustine, 1957)
Although popular newspapers and
magazines (e.g. Scientific American, 2009) unabashedly and
religiously promote an Earth-based abiogenesis, no one has
demonstrated or proved that life can be produced from non-life.
"Abiogenesis" is not even a true
scientific theory, but should instead be considered a speculative
hypothesis devoid of fact-based scientific support.
Even the
hypothesis of an Earthly "RNA World" where the first living things
are said to have had an RNA-based genome (Woese 1968), is fatally
flawed, as demonstrated by the reproductive strategy of viruses,
whose RNA-based genomes require the DNA of a living host.
By
contrast, the maxim: only life can produce life, has never been
discredited.
Certainly, if given billions of years of time where all the
essential ingredients and conditions are present, it is possible
life may have begun in a nebular cloud or on an ancient world whose
chemistry is different from Earth (Joseph and Schild 2010a,b).
However, there is no evidence to support
the belief that life on Earth originated from non-life. Given the
complexity of a single-celled organism and its DNA, the likelihood
that life on Earth began in an organic soup is the equivalent of
discovering a computer on Mars and claiming it was randomly
assembled in the methane sea.
Therefore, life on Earth must have also originated from life which
arrived her from other planets, and this theory is supported by
considerable evidence reviewed here, and in companion papers which
details the genetic evidence (Joseph 2009; Joseph and Schild 2010b;
see also Sharov, 2006, 2009).
This does not mean to argue that life
has no origins.
In an infinite universe and given
infinite opportunities and infinite combinations of the necessary
ingredients, it can be concluded that life may have arisen more than
once and in more than one location long before the creation of
Earth, our solar system, or this galaxy (Joseph 2010; Joseph and
Schild 2010a,b).
The descendants of these life forms were dispersed
throughout the cosmos via panspermia, and eventually fell to Earth.
"Panspermia" the theory that life on Earth came from space, comets,
or other planets, has an ancient history (Curd 2007), and has been
championed by esteemed scientists including,
-
Lord Kelvin (Thomson 1881)
-
von Helmholtz (1872)
-
Sir Fred Hoyle and Chandra
Wickramasinghe (Hoyle & Wickramasinghe 1993, 2000)
-
Nobel Laureates Svante Arrhenius
(1908) and Francis Crick (1981)
Arrhenius proposed that spores journey
through space propelled by photons.
Hoyle and Wickramasinghe have identified
comets and cosmic dust as a likely life delivery source. Crick
theorized that highly intelligent extra-terrestrial life evolved on
a planet much older than our own, and proposed purposeful
contamination which he termed "directed panspermia."
In a series of published video lectures which have been viewed
online (in total) millions of times, and in a series of scientific
articles published by this author and colleagues (Joseph 2000, 2008,
2009a,b, 2010; Joseph and Schild 2010a,b; Joseph and Wickramasinghe
2010) it has been proposed that living creatures arrived on Earth
contained in comets, asteroids, and planetary debris, the remnants
of planets shattered by a supernova.
There is in fact evidence that Earth is
a remnant from another solar system, a rogue planet which was
ejected prior to supernova (Joseph and Schild 2010a). If correct,
then microbial life may have been present in and on this planet long
before the birth of our solar system and may have subsequently been
seeded with additional life beginning over 4.5 billion years ago.
Microbes can form spores, become dormant and are adapted for
surviving the hazards of space (Horneck et al. 2002; McLean and
McLean 2010; Nickerson et al., 2004; Nicholson et al. 2000; Osman et
al., 2008; Szewczyk et al., 2005; Wilson et al., 2007), and spores
can return to life even after hundreds of millions of years
(Vreeland et al. 2000).
Microfossils and evidence of past
microbial life have been detected in fifteen carbonaceous chondrites
(discussed in section 11) which originated outside this solar
system, and possibly on other planets. Most have been impacted by
supernova. Thus, it is proposed that when planets were ejected from
solar systems during the red giant phase prior to supernova, and
debris produced during supernova contained living spores, and some
of this material fell to Earth and helped to form this planet, which
in turn may have long ago been ejected from a dying solar system.
Independent studies have provided evidence indicating life may have
been present on Earth, fractionating and synthesizing carbon,
throughout the Hadean eon, during the periods of heavy bombardment,
which extends from 3.8 billion years (BY) to the creation of this
planet 4.6 BY ago.
In 1978, the presence of microfosils resembling yeast cells and
fungi, was discovered in 3.8 BY old quartz, recovered
from Isua, S.W. Greenland (Pflug 1978).
Further evidence of biological including
photosynthesizing activity in these ancient rock formations is
indicated by the high carbon contents of the protolith shale, and
the ratio of carbon isotopes in graphite from metamorphosed
sediments dating to the same period (Rosing, 1999, Rosing and Frei,
2004).
Carbon-isotope evidence for life has also been found in
Quartz-pyroxene rocks on Akilia, West Greenland dated to 3.8 BY
(Manning et al. 2006; Mojzsis et al. 1996). Some of this evidence
was discovered within a phosphate mineral, apatite, which included
tiny grains of calcium and high levels of organic carbon; the
residue of photosynthesis, oxygen secretion, and thus biological
activity.
These "biological fingerprints" from 3.8 BY were created during a
period known as the "Late Heavy Bombardment" (Schoenberg et al.
2002) when the Earth, the moon, and other planets were pummeled with
debris that may have harbored complex life.
However, that bombardment began following the creation of this solar
system, causing the surface of the Earth to melt and form new rocky
layers. Thus additional evidence for life has been discovered in the
planet's oldest rocks, i.e. those which formed during the early
bombardment phase. These include the discovery of banded iron
formations in northern Quebec, Canada, consisting of alternating
magnetite and quartz dated to 4.28 BY, and which may be associated
with biological activity (O'Neil et al. 2008).
In addition, microprobe analyses of the
carbon isotope composition of metasediments in Western Australia
formed 4.2 BY revealed very high concentrations of carbon 12, or
"light carbon" which is typically associated with microbial life (Nemchin
et al. 2008).
Moreover, microfossils have been discovered in
ALH84001, a meteor
from Mars (McKay et al 1996) variably dated from 4.5 BY (Jagoutz,
1994; Nyquist et al., 1995) to 4.0 BY (Ash et al. 1996) to 3.8 BY (Wadhwa
and Lugmair 1996). This is a time period when both planets were
undergoing a heavy bombardment (Ash et al., 1996; Schoenberg et al.
2002).
Life may have been present from the very beginning when the Earth
was being pounded by stellar debris. As only life can create life,
this indicates that life on Earth may have arrived within that
debris.
Thus, life on Earth came from other
planets.
2. Red Giants,
Supernova & the Dispersal of Life
It is generally believed that our sun was created within a nebular
cloud produced by a supernova nearly 5 billion years ago.
According
to conventional wisdom, a protoplanetary disc formed from the
remnants of the nebular cloud surrounding the new sun, thereby
giving rise to the planets of this solar system (Greaves 2005; van
Dishoeck 2006). However, these planets, including Earth, may have
been remnants, or even rogue planets, ejected from the dying solar
system prior to supernova.
Microbes which took up residence on Earth during the Hadean period
also have as their likely source the parent star and its planets.
These microbes may have been dispersed in two stages.
Using our own solar system as an example (Schroder & Smith 2008)
when the parent star became a red giant, the accelerating power of
its solar winds would have blown away the life-sustaining
atmospheres of its planets which included airborne microbes,
creating a nebular cloud at the far edges of the dying solar system.
The parent star may have lost between 40% to 80% of its mass before
exploding (Kalirai, et al. 2007; Liebert et al. 2005; Wachter et al.
2008) and its planets would have significantly increased their
orbital distances and may have been ejected from its solar system
prior to supernova.
Thus the supernova may have shattered but
probably did not atomize all its planets. What would become Earth,
therefore, may have originated as an expelled planet which came to
reside in that nebular cloud.
And deep within this debris which
became part of the nebular cloud, innumerable microbes may have
continued to flourish.
3. Red Giant,
Solar Wind, & Wind Borne Microbes
Distinct species of over 1,8000 different types of bacteria and
other microbes thrive and flourish within the troposphere, the first
layer of the Earth's atmosphere (Brodie et al. 2007).
Air is an
ideal transport mechanism and serves as a major pathway for the
dispersal of bacteria, virus particles, algae, protozoa, lichens,
and fungi including those which dwell in soil and water.
Microorganisms and spores have been recovered at heights of,
-
40 km (Soffen
1965)
-
61 km (Wainwright et al., 2010)
-
up to 77-km (Imshenetsky,
1978)
These include Mycobacterium and Micrococcus, and fungi Aspergillus niger, Circinella muscae, and Penicillium notatum 77-km
above the surface of Earth (Imshenetsky, 1978).
Moreover, due to
tropical storms, monsoons, and even seasonal upwellings of columns
of air (Randel et al., 1998), microbes, spores, fungi, along with
water, methane, and other gases may be transported to the
stratosphere.
Hence, it can be readily assumed that microbes not
only flourish in the troposphere, but are commonly lofted into the
stratosphere (Wainwright et al., 2010).
Between September 22- 25, 1998, and as detected and measured by
NASA's Ultraviolet Imager aboard the Polar spacecraft, a series of
coronal mass ejections (CME) and a powerful solar solar wind created
a shock wave which struck the magnetosphere and the polar regions
with sufficient force to cause oxygen, helium, hydrogen, and other
gases to gush from the Earth's upper atmosphere into space (Moore
and Horwitz, 1998).
Normally the pressure is around 2 or 3 nanopascals. However, when the CME struck on Sept. 24, the pressure
jumped to 10 nanopascals. Thus it could be predicted that some
airborne microbes may have also been swept away.
Normally, such creatures might be too heavy to be lofted into space.
But under red giant conditions, it can be predicted that the solar
wind would strike with sufficient force to strip away atmosphere,
water molecules, surface dust (Schroder & Smith, 2008), along with
air-born bacteria, spores, fungi, lichens, algae, and other
microbes.
As the parent star entered the red giant phase, the outer layer
would have increased in size and the sun would lose mass at an
accelerated rate, swept away by an increasingly dense and clumpy
solar wind which streamed into space and buffeted its planets.
The
wind was likely accompanied by
Alfven waves which interacted with,
heated, and accelerated the solar wind (Suzuki 2007).
A nebular cloud would form from the ejecta, the inner layers (facing
the remnant heat from the supernova) would be warmer than the outer
layers (see discussion: Ehrenfreund and Menten 2002; Jansen et al.,
1994; Yamamato 1985; Yurimoto and Kuramoto 2004).
Nebular clouds,
along dust particles, would also provide protection against
radiation (Clayton, 2002; Flanner et al., 1980; Herbst and Klemperer
1973; Nishi et al., 1991; Prasad, and Tarafdar 1983).
As based on
related studies (Horneck et al. 2001a, b, 2002, 1994; Mitchell and
Ellis 1971; Nicholson et al 2000), spores would likely survive under
these conditions.
Water and a wide array of organics have been detected in nebular
clouds including polycyclic aromatic hydrocarbons and possibly the
amino glycine (Ehrenfreund and Menten 2002; Ehrenfreund and Sephton
2006; Gomez et al. 2008; Kaiser 2002; Schutte 2002; Snyder, 2004;
Stochel et al., 2009).
Some of this material is abiotic and may have
been produced within the molecular cloud.
However, some of the
organic molecules are larger than would be expected given the low
range of temperatures and pressures within these clouds. Thus, the
large organics may be biological and evidence of life such as that
dispersed by pre-supernova solar winds.
These findings also
strengthens the possibility that life may have originated in, or
currently dwells in nebular clouds.
4. Microbes
Are Pre-adapted for a Journey In Space
Many species of microbe have evolved the ability to survive a
violent hypervelocity impact and extreme acceleration and ejection
into space including:
-
extreme shock pressures of 100 GPa
-
the frigid
temperatures and vacuum of an interstellar environment
-
the UV rays,
cosmic rays, gamma rays, and ionizing radiation they would
encounter
-
the descent through the atmosphere and the landing
onto the surface of a planet
(Burchell et al. 2004; Burchella et al.
2001; Horneck et al. 2001a.b, Horneck et al. 1994; Mastrapaa et al.
2001; Nicholson et al. 2000, 2004; Mitchell and Ellis 1971).
For
example, a single dormant microbe was recovered from a lunar camera
which had set on the moon for 3 years and completely exposed to
radiation and freezing and broiling temperatures (Mitchell and Ellis
1971), whereas viable organisms, including the nematode, Caenorhabditis elegans (Szewczyk et al., 2005) and Microbispora sp
(McLean et al., 2006) survived the explosion and reentry of the
space Shuttle Columbia in 2003.
In fact, under space-flight
conditions of microgravity, viability may even increase and
microorganisms can actually thrive ( Nickerson et al., 2004; Wilson
et al., 2007).
As will be detailed in Section 8 microbes are pre-adapted for
traveling through space and living in almost every conceivable
environment (Section 7), and it can be assumed they would not have
evolved these capabilities if their entire ancestral and genetic
history had been confined to Earth and the conditions of this world.
5. Low Mass
Parent Star: Implications for Life
Given the paucity of evidence for nearby stars the same age as the
sun, it could be assumed only a few protostars may have been
produced by the supernova of the parent star.
Thus, the parent star
may have been only a few solar masses larger than the sun. This
assumption is supported by isotopic analysis of the Murchison
meteorite which is laden with microfossils and other indices of life
(Section 11).
Measurements of silicon carbide (Werner et al. 1994; Nittler & Hoppe
2005) and presolar SiC grains (Savina et al. 2003) from the
Murchison indicates that the grains and silicon are most likely the
residue of or were produced secondary to a supernova.
Supernova also
impacted a number of other carbonaceous chondrites (Birck, 2004)
which contain microfossils, including the Orgueil (Jadhav et al.
2006, 2007; Jadhava et al. 2006), Allende (Elgoresy and Ramdohr,
1980), Efremovka, and Ivuna (Shukolyukov and Lugmaira, 2006).
An analysis of ubiquitous of FE and NI carbides in the rims of
magnetite and the carbide grains within the Murchison (Brearly 2003)
indicates oxidation within the parent body of the meteor, which
could have been a much larger body such as a planet or planetesimal
(Ehrenfreund et al. 2001). An analysis of the presolar SiC grains
and other isotopes, indicates it was impacted by the supernova of a
carbon rich intermediate mass star that was between 1.5 to 3 solar
masses (Savina et al. 2003).
Planets have been detected orbiting intermediate mass stars (Lovis
and Mayor 2007).
Thus, the Murchison and the other carbonaceous chondrites impacted by supernova, may be a remnant of the parent
star's solar system, though this can't be determined at this time.
As only the estimated mass of that star is available and there is no
information on nearby stars at the time of supernova, a Hertzsprung-Russell
diagram cannot be applied to determine the age of the parent star at
the time of supernova. However, based on the estimated ages and
lifetimes of other intermediate mass stars (Pillitteri and Favata
2008) it can be estimated that a parent star of between 1.5 and 3
solar masses, was at least 1 billion to 3 billion years in age
before it entered the red giant phase.
Using the Earth as an
example, this is enough time for microbial life to flourish on its
planets, and for more complex life forms to begin to evolve.
6. Red Giants,
Supernova & Rogue Planets
The surface temperature of a red giant prior to supernova is much
lower than that of our sun.
For example, although over 2000 times as
large,
VY Canis Majoris is only 3,500 K, compared with the 5,778 K
of our sun (Monnier et al. 1999; Wittkowski et al. 1998). It is
unlikely an intermediate mass star which becomes a red giant would
have a surface temperature greater than our sun.
Therefore, microbes
living on planets circling at a sufficient distance would not be
unduly heated, particularly as these planets would have increased
their distance from the central star as it lost mass and expanded in
size.
The kinetic energy of an orbiting planet is half the energy of its
escape velocity. Planets as well as the central star exert
gravitational effects on one another (Gladman 2005). A star loses
from 40% to 80% of its mass during the red giant phase (Kalirai et
al. 2007; Liebert et al. 2005; Wachter et al. 2008). Therefore its
gravitational influences would be lessened.
Thus, planets that had
occupied an Earth-like habitable zone would have begun to increase
their distance from the parent star as it lost mass and expanded in
size (Schroder and Smith 2008). If these planets were larger than
the Earth (and depending on other parameters) they would likely be
expelled from the solar system prior to supernova (Schroder and
Smith 2008).
One planet, having a mass 4.2 times that of the Earth, has been
detected orbiting a main sequence star,
HD 40307, located 42
light-years away towards the southern Doradus and Pictor
constellations (Mayor et al. 2009).
Another, planet having a mass 5
times and a radius 1.5 times that of the Earth was discovered
orbiting the "habitable zone" of
Gliese 581 in the constellation
Libra, and with an estimated surface temperature between 0oC and 40oC (Udry et al. 2007)
- a temperature range amenable to even complex
creatures.
As these "super Earths" appear to be common (Mayor et al. 2009),
similar "super Earths" may have orbited the parent star, but may
have been expelled prior to supernova and during the early red giant
phases.
Thus, when the parent star exploded, although some of its
planets may have been shattered, it is unlikely they would have been
atomized if they had been ejected.
Consider again the Murchison. An analysis of the isotopic
composition of silicon carbide grains, indicates impact by shock
waves from a supernova of a star which has lost almost all of its
hydrogen mass (Pellin et al. 2002), indicating its gravitational
influences were significantly reduced.
These findings, coupled with
results from shock-recovery experiments performed on insoluble
organic matter within the Murchison (Mimura et al. 2007) are also
consistent with the proposal that its parent body was expelled from
the solar system prior to supernova, and was then impacted following
supernova, perhaps while drifting within a planetary nebula.
7. Life
Thrives in Extreme Environments
Habitable planets which are expelled and shattered might continue to
harbor life.
Communities of hyperthermophiles have been recovered
from geothermally heated rocks 2,500 to 3500 meters beneath the
surface of the Earth (Boone et al. 1995; Setter 2002), and aside 400oC rock chimneys at depths of 4000 meters (Setter 2002), at the
bottom of the ocean where pressures are 9000 pounds per square inch.
Baccilus infernus, thrives at depths of 2,700 meters where the
weight and pressure is 300 x that of the surface and where
temperatures may exceed 117oC (Boone et al. 1995). Hyperthermophiles
continue growing even in 100oC boiling water (Setter 2002) and they
can survive without oxygen (Setter 2002), liberating chemicals and
minerals for energy.
Microorganisms have also been recovered from cores 400 meters deep
in the Canadian arctic (Gilinchinsky 2002). Viable cells dated to 3
million years have been recovered in northeast Siberia.
According to Gilinchinsky (2002),
"The permafrost can maintain life incomparably
longer than any other known habitats" and "contain a total microbial
biomass many times higher than that of the soil."
"The permafrost
community have overcome the combined action of extremely cold
temperature, desiccation, and starvation" and "life might be
preserved in permafrost conditions for billions years."
It can be predicted that a substantial number of microbes such as
those normally dwelling deep beneath the surface, near volcanic
substrates, or frozen within the ice, could easily survive if the
host planet were shattered or ejected from the solar system, and
would do so under localized conditions little different from those
prior to a supernova.
8. Radiation
Resistance Microbes
The shock wave of a supernova would accelerate electrons, protons,
and ions and heat the interstellar medium.
Incredible amounts of
energy would be released in the form of radioactive isotopes, free
electrons, X-rays and gamma rays. However, a significant number of
microbes would be unaffected.
When subjected to life neutralizing conditions, microbes and other
single celled creatures can form spores and become dormant. Further,
a spore can form a highly mineralized core enclosed in heat or cold
shock proteins which wrap around and protect them (Marquis and Shin
2006).
They will also saturate their DNA with acid
soluble proteins
which alters the enzymatic and chemical reactivity of its genome
making it nearly impermeable to harm (Setlow and Setlow 1995).
"In the dormant stage a spore has no metabolism and resists cycles
of extreme heat and cold, extreme desiccation including vacuum, UV
and ionizing radiation, oxyidzing agents and corrosive chemicals"
(Nicholson et al. 2000). Space experiments and the
Long Duration
Exposure Facility Mission have shown that bacteria and fungal spores
can easily survive the vacuum of space and constant exposure to
solar, UV, and cosmic radiation with just minimal protection (Horneck
1993; Horneck et al.1995; Mitchell and Ellis, 1971).
However,
survival rates increase significantly from between 30% to 70% if
coated with dust, or embedded in salt or sugar crystals (Horneck et
al. 1994). In fact, on Earth, bacterial spores embedded in salt
crystals dated to 250 million years (Satterfield et al. 2005),
survived and were brought back to life (Vreeland, et al. 2000).
Viable cells lofted into the vacuum of space in the absence of a
protective environment would also react to the absence of water in
space by becoming "freeze dried" and dormant (Setlow, 2006). Under
these conditions these microbes develop heightened resistance to
radiation and extremes in temperature (Sunde et al., 2009).
Not just spores, but lichens, fungi and algae survive exposure to
massive UV and cosmic radiation and the vacuum of space (Sancho et
al. 2005).
Lichens show nearly the same photosynthetic activity
before and after space flight, and multimicroscopy investigation
revealed no detectable ultrastructural changes in most of the algae
and fungal cells (Sancho et al. 2005).
Microfossils resembling fungi were discovered in 3.8 million year
old quartz, recovered from S. W. Greenland (Pflug 1978); a time
period corresponding to the "late heavy bombardment." And vast
colonies of algae were building
stromatolites by 3.5 billion years
ago. Thus, these fungi and algae could have survived a journey
through space and may have been deposited on the Earth.
Although the full spectrum of UV rays is deadly against spores, the
likelihood of a direct hit, even if unprotected while traveling
through space is unlikely. Moreover, just a few meters of surface
material offers more than sufficient protection for those buried
deep inside (Horneck et al. 2002).
Microbes were recovered from a camera that had sat on the moon for
nearly 33 months, directly exposed to the vacuum of space, constant
radiation, an average temperature of 20 degrees above absolute zero,
and without nutrients, water or energy source (Mitchell and Ellis,
1971).
When brought back to Earth they recovered and began to
reproduce.
High-energy radiation or particles from extraterrestrial space (HZE)
that strike a meteor, asteroid, comet, or lunar camera, may create
secondary radiation.
Studies have shown that as the thickness of
surrounding material increases beyond 30 cm, the dose rate and
lethal effects of heavy ions, including secondary radiation,
depreciates significantly (Horneck et al. 2002). B. subtilis spores
can survive a direct hit even though HZE particles can penetrate
thick shielding (Horneck et al. 2002).
Moreover, many species of microbe can withstand X-rays and atomic
radiation, and are radiation resistant.
These include:
-
Deinococcus
radiodurans
-
D. proteolyticus
-
D. radiopugnans
-
D. radiophilus
-
D. grandis
-
D. indicus
-
D. frigens
-
D. saxicola
-
D. marmola
-
D. geothermalis
-
D. murrayi
These species can rebuild their genomes
even if shattered by radiation (Lovett, 2006) and the same is true
of yeast (Scheifele and Boeke 2008).
Microbes and spores are so small that even when bombarded with
photons and deadly gamma and UV rays the likelihood they would be
struck is infinitesimally minute. Estimates are that a spore may exist
in space for up to a million years in space before it may be struck
(Horneck et al. 2002). Even after 25 million years in space, a
substantial number of spores would survive if shielded by 2 meters
of meteorite (Horneck et al. 2002).
Likewise, those microbes, algae, fungi, and lichens dispatched from
the surface of their home planets by an increasingly powerful solar
wind prior to supernova, and which became part of the growing
nebular debris field, would have been protected.
The central layers
of a molecular cloud shields against deadly gamma and cosmic rays
which are deflected by dust, ice and debris (Clayton, 2002; Ehrenfreund and Menten 2002; Herbst and Klemperer 1973; Nishi et
al., 1991; Prasad, and Tarafdar 1983), and are sufficiently warm
that ice evaporates (van Dishoeck 2006).
Further, many species of bacteria and microbes form colonies. Those
on the outer layers, if killed, create a protective crust, blocking
out radiation and protecting those in the inner layers from the
other hazards of space (Nicholson et al. 2000). Therefore, be they
buried within rock, ice, or some other stellar material, colonies of
living microbes could provide their own protection.
Clearly, these extraordinary life-sustaining capabilities were
acquired after repeated and prolonged ancestral experiences within
an interstellar environment involving journeys through space and
from planet to planet.
Microbes are perfectly adapted for a life in
space and could not have evolved these capabilities if their entire
ancestral and genetic history had been confined to a life on Earth.
9. Planet
Formation, Rogue Worlds, & Spore Survival
A supernova creates tremendous shock waves, shattering its planets,
and expelling most of the star and remaining planetary debris into
the surrounding interstellar medium.
This debris eventually becomes
part of the surrounding nebular ring created by the solar winds,
planetary atmospheres, and expelled mass of the dead star (Greaves
2005, van Dishoeck 2006).
Over hundreds of thousands of years and in response to cosmic shock
waves and radiant energy produced perhaps by nearby exploding stars,
the debris within these clouds begins to clump together, generating
tremendous amounts of energy as they grow larger and denser and
become enveloped by hydrogen gas. Add to this the influences of
Quasars and black holes which direct streams of hydrogen gases into
nebular clouds and which appear to envelop these super-hydrogen gas
giants (Joseph and Schild 2010a).
Therefore, as these proto-stars
grow an ever denser hydrogen atmosphere, they finally ignite, such
that dozens, hundreds, even thousands of proto-stars may be created
(Hester et al. 2004). According to the most conservative estimates
it could take a hundred million years for a new sun to form (Montmerle
et al. 2006).
These young stars are surrounded by a debris field,
perhaps containing planetary fragments or even rogue planets. The
debris field eventually flattens and forms a
protoplanetary disc.
The inner layers of a protoplanetary disc are not easily penetrated
by UV photons, gamma and cosmic rays or radiation from the sun due
to dust and debris (van Dishoeck 2006).
The central and inner layers
(facing the proto-star) provide a protective environment for
microbial survivors, and are sufficiently warm (Glassgold et al.,
2004; Kamp and Dullemond 2004) that ice evaporates and water vapors
form (Eisner 2007; van Dishoeck 2006).
Planet formation proceeds at a relatively rapid pace, from a few
hundred years for a small rocky planet the size of the Earth to one
million years for Jupiter and Saturn-sized gas giants (Kokubo and
Ida 2002) depending on the initial disk mass. Computer models and
observations of planet-formation have given us an understanding of
the mechanisms (Kokubo and Ida 2002; Raymond et al. 2007).
Within
just a few thousand years the remnants of the supernova and the
nebular cloud which surrounded the proto-sun, begin to flatten out
into a swirling circular proto planetary disk. According to
conventional wisdom, after just a few spins around the new
proto-star, islands of debris begin to collide and clump together
forming moon-like planets, increasing in size by accretion (Goldreich
et al. 2004).
However, the only way a planet can grow by accretion
is if the planet was already quite large to begin with and then
accumulated mountains of much smaller debris.
According to the most conservative estimates, if mechanisms of
accretion are very slow, it could take up to a million years for a
massive solid planetary core to form. Then it would quickly snow
ball in size through clumping and as debris continued to crash into
it (Montmerle et al. 2006).
Yet another explanation is that when a star supernovas, it ejects
molten iron into these nebular clouds. Therefore, planets begin to
form when debris comes into contact with and then sticks to the hot
iron which becomes a planetary core (Joseph and Schild 2010a). Then
there are those rogue planets which were ejected prior to supernova.
Therefore, some solar systems may acquire fully formed or broken and
shattered planets which grow by accretion after they are captured by
the new proto-star.
Greaves et al. (2008) discovered a protoplanet which she reports
(National Geographic, 2008) was created 2,000 years ago, circling a
young star HL Tau, which is 100,000 years old. Could a planet form
so quickly from debris crashing together?
It has been estimated that the core of the Earth was rapidly formed
from chondrites and then underwent rapid accretion within 10 million
years such that our planet was formed approximately 30 million years
after the origin of our solar system (Jacobsen, 2005).
Chondrites,
however, are the remnants of other planets.
Planetary cores therefore, may be comprised of the remains of the
shattered planets which had been expelled from the solar system of
the parent star prior to supernova. However, entire planets would
have also been ejected. This would explain why full formed planets
were already crashing into each other during and soon after the
formation of this solar system.
Jacobsen (2005) estimates that
within 30 million years after the formation of the solar system,
Earth was struck by a Mars-sized planet.
These shattered and intact rogue planets could have harbored spores,
microbes, viruses, bacteria, lichens, yeast, and algae.
If correct,
then it could be predicted that the descendants of microbes whose
genomes are alien to the surface of our planet, and which were
buried by accumulating stellar debris should be discovered miles
beneath the Earth and below the subfloor of the ocean; and this
prediction has been born out (Biddle et al., 2008; Chivian et al.,
2008; Doerfert et al., 2009; Moser et al., 2005; Gohn et al., 2008;
Hinrichs et al., 2006; Sahl et al., 2008).
Consider for example, the
bacterium,
Desulforudis audaxviator, discovered 2.8-kilometers (1.74
miles) beneath the surface.
Genomic analysis of its 2,157
protein-coding genes indicates this species,
"is capable of an
independent life-style well suited to long-term isolation from the
photosphere deep within Earth's crust and offers an example of a
natural ecosystem that appears to have its biological component
entirely encoded within a single genome."
(Chivian et al., 2008)
The
genome of D. audaxviator indicates it has never been exposed to
sunlight, obtains its nourishment from non-biological sources, and
can form spores.
The presence of species miles beneath the surface of our planet
suggests they were deposited there, encased in planetary debris, as
the Earth was formed. However, this would also imply that a smaller
Earth was captured by this solar system and then grew by accretion.
Therefore, the core of every planet in our solar system may be
comprised of the remains of the shattered planets which had been
expelled from the solar system of the parent star prior to
supernova. These shattered rogue planets could have harbored spores,
microbes, viruses, bacteria, lichens, yeast, and algae.
Survival of these broken planets, and their presence within the
protoplanetary disc would explain why Uranus, Mars, the Earth, and
Mercury may have been struck by wayward worlds during the early
years of solar system formation (Gladman 2005; Goldreich et al.
2004; Nimmo et al. 2008).
For example, around 4 billion years ago, a
Mars-sized planet may have hit the Earth with so much force that the
ejected mass may have come to form the moon (Belbruno and Gott III
2005; Poitrasson et al. 2004, Rankenburg et al. 2006).
Around 4 billion years ago, the northern plains of Mars was gutted
by a planet-sized body which left an elliptical depression 6,600
miles long and 4,000 miles wide (Andrews-Hanna et al. 2008). The
planet Mercury may have also suffered a collision which left a
titanic impact basin. Uranus was apparently struck so hard by an
Earth-sized body that its rotation axis tilted sideways, nearly into
the plane of its revolution about the Sun (Bergstralh et al. 1991).
Thus, there is considerable evidence that planet-sized objects were
careening through the solar system during its early stages of
formation and in directions and trajectories different from the
other planets. It also appears that the sun and its planets had
become established within a 100 million years.
A 100 million years is more than enough time for any surviving life
forms contained in the remnants of the parent star's shattered
planets or the nebular cloud or the protoplanetary disk to find safe
harbor within a new world made up of this debris. Some microbes
become dormant and can reawaken even after 250 million years
(Vreeland et al. 2000).
In fact "living bacteria" have been
discovered and "isolated from salt deposits from the Middle
Devonian, the Silurian, and the Precambrian" making some of them
over 600 million years in age (Dombrowski 1963).
Thus microbes may survive from 250 million to 600 million years.
However, only one microbe had to survive, and once on Earth could
cover the planet in bacterial offspring within a few months.
10.
Extraterrestrial Contamination of Earth by Microbes
Experiments have shown that microbes can survive the shock of a
violent impact casting them deep into space (Mastrapaa et al. 2001;
Burchell et al. 2004; Burchella et al. 2001).
Further, a substantial
number could easily survive the descent to the surface of a planet (Burchella
et al. 2001; Horneck et al. 2002), even following high atmospheric
explosions, i.e. the Columbia space shuttle explosion (Szewczyk et
al., 2005) and rentry speeds of 9700 km h-1 (McLean et al., 2006).
When meteors strike the atmosphere, they are subjected to extremely
high temperatures for only a few seconds. If of sufficient size, the
interior of the meteor will stay relatively cool, with the surface
material acting as a heat shield. Thus the heat does not effect the
material uniformly.
The interior may never be heated above 100 C (Horneck
et al. 2002), whereas spores can survive post shock temperatures of
over 250 C.
11. Meteors &
Microfossils
Microbial fossils have been found in fifteen carbonaceous chondrites
including the,
-
Murchison (Claus and Nagy 1961; Hoover 1984, 1997; Pflug
1984)
-
Ivuna (Claus & Nagy 1961)
-
Orgueil [Hoover 2004; Nagy et
al. 1961,1963a,b; Claus and Nagy 1961)
-
Allende (Folk and Lynch
1997; Zhmur and Gerasimenko 1999)
-
Efremovka (Zhmur and
Gerasimenko 1999; Zhmur et al. (1997)
The fossilized impressions of nanobacteria, extremophiles, and colonies resembling cyanobacteria
have been detected by several independent investigators and NASA
scientists.
Organic material and biogenic hydrocarbons believed to have been
produce by extraterrestrial creatures, and organized elements and
cell structures that resemble fossilized algae were identified as
indigenous to the Orgeuil (Claus & Nagy 1961; Nagy et al. 1962; Nagy
et al. 1963a,b,c).
Smooth filamentous and spherical skins
surrounding grains of inorganic material were discovered, and many
were doubled like the walls of biological cells. Some of the skins
resembled microscopic fungi.
Further study of
the Orgueil and
Ivuna meteorites using an electron
probe and electron microscope, revealed the presence of microfossils
(Nagy et al., 1962, 1963a,b), some of which were very similar to
purple photosynthetizing bacteria belonging to the species
Rhodopseudomonas rutilis (Pflug 1984).
Additional confirmation was
provided by Richard Hoover of NASA, who discovered, using NASA's
Field Emission Scanning Electron Microscope, fossilized colonies
resembling
cyanobacteria (Hoover 1984).
These fossils were found in
a freshly fractured, interior slice of the Orgueil meteorite, making
it almost impossible they are due to contamination.
Pflug (1984), discovered virus particles and clusters of an
extensive array of microfossils within the Murchison meteorite,
similar to terrestrial bacteria such as methanogens and
Pedomicrobium, a flowering bacteria which feeds on metals.
Pflug
(1984) used acid to dissolve the mineralized portions and identified
numerous fossil like structures nearly identical to those found in
ancient terrestrial rock and iron banded formations in Gunflint
Minnesota --these formation extend backwards in time to 4.2 billion
years ago.
These results were confirmed by Hoover (1997) who discovered
fossilized bacteria deep within the Murchison meteorite which
resemble colonies of living cyanobacteria.
According to Dr. Hoover
(1997),
"the fossils were seen in freshly broken pieces of the
meteorite so the chance that they were earthly contaminants is low.
The chemical evidence around the microfossils is most readily
explained as the result of biological activity."
As summed up by Dr Hoover (1997):
"The Murchison forms represent an
indigenous population of the preserved and altered carbonized
remains (microfossils) of microorganisms that lived in the parent
body of this meteorite at diverse times during the last 4.5 billion
years."
Fossils of microorganisms, cyanobacteria and coccoid bacteria similar
to the Synechococcus genera were found inside the Efremovka
meteorite (Zhmur and Gerasimenko 1999).
Zhmur et al. (1997)
conducted a,
"comparative analysis of bacteriomorphic structures from
the carbonaceous meteorites Murchison, Efremovka, and Allenda" and
the "morphology of microorganisms of modern and ancient
cyanobacterial community."
They concluded that fossils found on
these three meteors are the,
"fossilized remnants of microorganisms.
The lithified remnants are tightly conjugated with the mineral
matrix, removing the possibility they are contaminants."
The Ivuna, Orgueil, Murchison, Allende, and Efremovka meteorites are
all carbonaceous chondrites.
Carbonaceous chondrites typically
contain a high abundance of water-bearing minerals, organics and
biologically related compounds (see e.g. Botta and Bada, 2002;
Hoover 2006; Sephton 2002).
Numerous independent research groups have detected the nucleobases
for DNA and RNA within carbonaceous chondrites, including adenine,
guanine, uracil, as well as melamine (Hayatsu, 1964; Hayatsu et al.
1968, 1975; Hayatsu et al. 1968; Folsome et al. 1971,1973; Lawless
et al. 1972; Van der Velden and Schwartz, 1977; Stoks and Schwartz
1979, 1981; Martins et al. 2008).
These organics are
extra-terrestrial in origin (Van der Velden and Schwartz 1977;
Martins et al. 2008) and were most likely produced biologically
(Hoover 2006).
As only life can produce life, these are not
pre-biotic.
Amino acids and nucleobases for DNA and RNA, including adenine,
guanine, alanine, glyciine and isovaline were also discovered in the
interior of the Orgeuil (Hayatsu 1964; Hayatsu et al. 1968; Folsome
et al. (1971, 1973; Lawless et al. 1972). Carbon isotopic
measurements demonstrated these acids were extraterrestrial and
originated in an environment with water and a high concentration of
organic carbon.
Thus, they are likely biological in origin.
The presence of DNA and RNA fragments are also an indication of
extraterrestrial life. The genomes of living creatures journeying
through space, can be fractured and broken if struck by radiation
(Dose et al. 1995).
Thus many meteorities contain DNA nucleotides
which may be the broken remnants of bacterial DNA.
The Murchison also contains an extensive array of organic compounds
including nitrogen bases and over 70 different amino acids and a
preponderance of left-handed aminos which are characteristic of life
(Cooper et al. 2001; Cronin et al. 1993).
These include guanylurea,
triazines, aliphatic amines, a pyrimidine, and purines, e.g.,
adenine, guanine, uracil (Hayatsu et al. 1975; Folsome et al. 1971,
1973; Stoks and Schwartz, 1979, Van der Velden and Schwartz, 1977,
Martins et al. 2008). Additional study found sugars, organic residue
and vesicles that had formed organic compounds (Dreamer 1985;
Dreamer and Pashley 1989; Lawless & Yeun, 1979; Yuen et al. 1984).
These include fatty acids similar to the albumin of egg yolk.
In addition, Hoover and colleagues (Hoover, 1997, 2006; Hoover and
Rozanov, 2003; Hoover et al. 1998, 2003, 2004) have examined a
number of additional carbonaceous meteorites (i.e., Acfer, Alais,
Dar al Gani Kainsaz, Karoonda, Mighei, Murray, Nogoya, Rainbow,
Tagish Lake) and have detected microstructures similar to
nanobacteria (50-400 nm) and spherical bodies (1 m-20 m) embedded
in the meteorite matrix, resembling cocci, chroococoid and
cyanobacteria.
Many of these meteors have been impacted by supernova (Birck 2004;
Jadhav et al. 2006, 2007; Elgoresy and Ramdohr, 1980; Shukolyukov
and Lugmaira 2006) and predate the origin of this solar system.
12.
Discussion: Contamination & the Origin of Earthly Life
Critics commonly dismiss all evidence of microfossils in meteors by
claiming contamination. Likewise, evidence for the presence of life
during the Hadean eon have also been attacked as due to
contamination.
However, claims of contamination are not proof of contamination. No
one has ever conclusively demonstrated this overwhelming body of
evidence, from numerous independent investigators, is in fact due to
contamination.
If these findings of microfossils are due to "contamination" then we
must ask: why does this "contamination" only occur in stony
meteorites? As reported by Hoover (2006), ten non-carbonaceous
meteorites were studied including iron meteorites. Not one was found
to contain microfossils or evidence of life.
Innumerable Earthly microbes feast on metals. It is not likely that
microbes from Earth would leave their fossilized signatures deep
within stony meteors, but avoid those consisting of silicates, irons
and other metals.
Iron meteorites are believed to have originated in the molten core
of a much larger body, and thus would never have been expected to
harbor life. By contrast, many chondrites, although linked to
comets, likely originated as part of the deep surface layers of a
planet or
planetesimal (Ehrenfreund et al. 2001) and are similar to
rocks found on the surface of the Earth.
As such chondrites and not
iron meteors would be expected to harbor life prior to crashing to
Earth.
For example, mineralogical and petro-graphic evidence and the
presence of carbonates and sulfates within these chondrites indicate
considerable aqueous activity (Fredriksson and Kerridge, 1988;
Bostrom and Fredriksson 1966; Kerridge, 1967) and possibly an
original environment similar to permafrost (Dufresne and Anders
1962).
When coupled with oxygen isotope data (Clayton and Mayeda
1984) it appears these chondrites were not formed in a nebular
cloud. In fact, as based on an analysis of the amino acids of the
Orgueil, Ivuna and Murchison meteorites it is possible that each
originated on a different planet (Ehrenfreund et al. 2001).
Given these different histories, chondrites would be expected to
have been infiltrated with microbes before they struck the Earth.
The same would not be expected of iron meteors. If due to
contamination, both types of meteors should contain microfossils and
they do not.
The question of contamination is not an argument against
extra-terrestrial life, but instead explains how life originated on
this planet: via contamination.
No one has ever demonstrated that Earthly life can be produced from
non-life, at least on Earth. The maxim: only life can produce life,
has never been discredited. Therefore, based purely on the facts and
scientific evidence, the only reasonable explanation is that the
first living creatures to appear on this planet were produced by
other living things which arrived on Earth safely encased in the
debris which was bombarding this world during the Hadean eon.
The evidence detailed in this paper provides a scientific
explanation for how life originated on Earth, and why there is
evidence of biological activity in banded iron formations dated to
4.28 billion years ago (O'Neil et al. 2008), within meta-sediments in
Western Australia formed 4.2 billion years ago (Nemchin et al.
2008), and thus why life appeared in the oldest rocks on Earth while
the planet was still forming.
Likewise, the presence of life within
extra-terrestrial debris accounts for the biological activity within
rock formation located in West Greenland, and the nearby Akilia
island dated to almost 3.9 billion years in age (Manning et al.
2006; Mojzsis et al. 1996) and in 3.8 million year old quartz,
recovered from Isua, S. W. Greenland, and which included
microfossils resembling yeast cells and fungi (Pflug 1978).
Independently obtained evidence of biological activity including
photosynthesis was also discovered in this area dated from the same
time period (Rosing 1999, Rosing and Frei 2004).
Furthermore, microbe infested meteors which bombarded the inner
planets during the Hadean era can also account for the evidence of
microbial life in a meteor from Mars (McKay et al 1996) which has
been variably dated from 4.5 to 3.8 BY (Ash et al. 1996; Jagoutz,
1994; Nyquist et al., 1995; Wadhwa and Lugmair 1996).
13. The Earth
is Not the Center of the Biological Universe
"Living things are specified with incredible delicacy and precision
down to the level of individual molecules and atoms. It is simply
impossible for a random mixture of relevant and irrelevant molecules
and atoms to individually sort themselves out, expell those which
are irrelevant, and then assemble those which remain thereby
giving life to non-life."
-Nobel Laureate, Francis
Crick (1981)
The question of origins has been debated for thousands of years.
According to the Greek philosopher, Anaximander (610 BC-546 BC),
life first emerged when watery soil was evaporated by the sun, and
there followed a progression of life forms which became increasingly
complex (Kahn 1994; Plutarch, Symposium 8).
Over the ages many
scientists have accepted this view as there is an intuitive appeal
to the claim that life came from non-life. And yet there is no proof
to substantiate this claim, and "intuition" like other beliefs
without factual foundation, is not science.
Yet other factors contribute to the widespread faith in abiogenesis
as an explanation for the origin of life on Earth, including
religion and the magical thinking characteristic of early childhood,
where inanimate objects such as toys, shadows, rocks, and so on, can
be endowed with life. Piaget (1955, 1958) referred to this
intuitive, egocentric stage of intellectual development as
"Preoperational."
Preoperational thinking dominates during the ages
of 2-4, when children believe the world revolves around them, and
where they assign living attributes and even personalities and
purposeful behavior to inanimate objects. It is difficult to
completely escape these influences, for the child is father to the
man.
Therefore, whereas the child saw him/herself as the center of the
universe and believed non-life could be endowed with life, many in
the scientific community would have us believe the Earth is the
center of the biological universe, where non-life gave rise to life;
and revolving around us, the non-living cosmos.
In this article no attempt is made to explain the origins of life.
However, given the incredible vastness and unknown age of the
universe, and the little blue dot we call Earth, isn't it reasonable
to assume life would have emerged somewhere in the cosmos long
before it appeared on this insignificant spec of dust? Certainly,
the world does not revolve around us, the Earth is not the center of
the solar system, and our planet is not at the center of the
biological universe.
The age of the universe is unknown, though speculation abounds.
Those believing in a Big Bang universe have provided a wide range of
birth dates over the last 80 years, ranging from 2 billion to over
20 billion years.
However, there is also substantial evidence
indicating the universe may be infinite (Joseph 2010), and in an
infinite universe, life has had infinite opportunities to arise from
infinite chance combinations of the necessary ingredients.
14. Conclusion
"If Life were to suddenly appear on a desert island we wouldn't
claim it was randomly assembled in an organic soup or created by the
hand of god; we'd conclude it washed to shore or fell from the sky.
The Earth too, is an island, orbiting in a sea of space, and living
creatures and their DNA have been washing to shore and falling from
the sky since our planets creation."
(Joseph, 2000)
Evidence for biological activity appears in the oldest rocks on
Earth, during a period of heavy bombardment while this planet was
forming. Biological activity in a meteor from Mars dates from the
same period.
Microfossils have been detected in fifteen carbonaceous chondrites,
almost all of which have been impacted by supernova, and several of
which may have originated on planets that predated the origin of
this solar system.
Our sun and solar system were created from the nebular debris
spawned by a red giant which exploded in a supernova, nearly 5
billion years ago. The sun and our solar system may have been
created within 100 million years of this explosion. Planets, such as
Earth, may have originated in the star system which gave birth to
our own, and then grew by accretion after becoming captured by the
new proto-star which would become the sun.
Spores can survive from 250 to 600 million years; which is more than
enough time to take up residence on planets made up of the debris
which pounded new Earth. Bacteria are perfectly adapted for
surviving the hazards of space, and could not have acquired these
abilities if their ancestral experience had been confined to Earth.
Life on Earth appeared while this planet was still growing by
accretion. There is no proof life can be created from non-life.
Certainly it is possible life may have first been generated on an
ancient world or in an environment with a chemistry completely
unlike the Earth. Nebular clouds are excellent candidates (Joseph
and Schild 2010a,b).
If the analysis of increasing genetic
complexity provided by Sharov (2006, 2009) is verified by others and
our genetic ancestry does lead to a life form which first achieved
life 10 billion years ago, then it also seems reasonable to assume
that the nature of the Milky Way galaxy at that time provided the
conditions necessary for the establishment of life; i.e. nebular
clouds.
If correct, then the sequence of stellar events described in
this paper could also be applied to the descendants of that first
life form, such that over the ensuing 10 billion years this galaxy
was seeded with life which originated in a nebular cloud.
The theory proposed in this paper also lends direct support to the
"life cloud" speculations of Sir Fred Hoyle (1957) and
Chandra Wickramasinghe (Hoyle and Wickramasinghe 1978) and the impressive
body of evidence Hoyle and Wickramasinghe (1993, 2000) have
marshaled for comets as celestial mechanisms which seed planets with
life.
However, the fact remains there is no evidence life on our planet
began in an organic soup via
abiogenesis.
As only life can produce
life, only
panspermia is a viable scientific explanation as to the
origin of Earthly life. The first life forms to appear on Earth were
produced by other living creatures who were likely encased in debris
from the shattered remnants of those planets that circled the parent
star nearly 5 billion years ago.
Life on Earth, came from other planets.
REFERENCES
Andrews-Hanna, J. C., Zuber, M. T.,
Banerdt, W. B. 2008. The Borealis basin and the origin of the
martian crustal dichotomy Nature 453, 1212-1215.
Arrhenius, S. 1908. Worlds in the Making. Harper & Brothers, New
York.
Ash R. D., Knott S. F., and Turner G. (1996) A 4-Gyr shock age
for a martian meteorite and implications for the cratering
history of Mars. Nature, 380, 57-59.
Augustine, St. 1957. City of God. Harvard University Press.
Becker, S. A. 1981. The evolution of intermediate-mass stars
from the zero-age main sequence to the base of the asymptotic
giant branch as a function of mass and composition.
Astrophysical Journal Supplement Series, vol. 45, p. 475-505.
Belbruno, E., Gott III, J. R. 2005. Where Did the Moon Come
From? The Astronomical Journal 129 1724-1745.
Bergstralh, J. T., Miner, E., Matthews, M. 1991. Uranus. Univ.
of Arizona.
Biddle, J. F., et al., 2008. Metagenomic signatures of the Peru
Margin subseafloor biosphere show a genetically distinct
environment. PNAS, 105, 10583-10588.
Boone, D R., Liu, Y., Zhao, Z. J., Balkwill, D. L., Drake, G.
R., Stevens, T. O., Aldrich, H. C, 1995. Bacillus infernus sp.
nov., an Fe(III)- and Mn(IV)-reducing anaerobe from the deep
terrestrial subsurface. International journal of systematic
bacteriology. 45(3):441-8.
Bostrom, K., Fredriksson, K. 1996. Surface conditions of the
Orgueil parent meteorite body indicated by mineral associations,
Smiths Misc. Coll 151, 1-39.
Botta, O., Bada, J.L., 2002. Extraterrestrial organic compounds
in meteorites. Surv. Geophys. 23, 411-467.
Brearley, A. J. 2003. Ubiquitous nanophase FE, NI Carbides in
Murchison fine-grained rims: possible reflects of nebular
Fisher-Tropsh reactions. 66th Annual Meteoritical Society
Meeting.
Burchella, M. J., Manna, J., Bunch, A. W., Brandob, P. F. B.
2001. Survivability of bacteria in hypervelocity impact, Icarus.
154, 545-547.
Burchell, J. R. Mann, J., Bunch, A. W. 2004. Survival of
bacteria and spores under extreme shock pressures, Monthly
Notices of the Royal Astronomical Society, 352, 1273-1278.
Brodie, E. L., DeSantis, t. Z., Parker, J. P. M., Zubietta, I.
X., Piceno, Y. M., Andersen, G. L. 2007. Urban aerosols harbor
diverse and dynamic bacterial populations PNAS. 104, 299-304.
Canup, R. N., Asphaug, E. 2001. Origin of the Moon in a giant
impact near the end of the Earth's formation Nature 412,
708-712.
Chivian, D., et al., 2008. Environmental Genomics Reveals a
Single-Species Ecosystem Deep Within Earth Science 322, 275-278.
Claus, G., Nagy, B. (1961) A Microbiological Examination of Some
Carbonaceous Chondrites. Nature 192, 594 - 596.
Clayton, R. N., Mayeda, T. K. 1984. The oxygen isotope record in
Murchison and other carbonaceous chondrites, Earth Planet. Sci.
Lett., 67, 151-161.
Clayton, R. N. 2002, Solar system: Self-shielding in the solar
nebula, Nature 415, 860-861.
Cooper, G., Kimmich, N., Belisle, W., Sarinana, J., Brabham, K.,
Garrel, L., 2001. Carbonaceous meteorites as a source of
sugar-related organic compounds for the early Earth. Nature 414,
879-883.
Crick, F. 1981. Life Itself. Its Origin and Nature. Simon &
Schuster, New York.
Cronin, J.R., Pizzarello, S., Epstein, S., Krishnamurthy, R.V.
1993. Molecular and isotopic.analysis of the hydroxyl acids,
dicarboxylic acids and hydrocarboxylic acids of the. Murchison
meteorite. Geochim. Cosmochim. Acta 57, 4745-4752.
Curd, P. (translator) 2007. Anaxagoras of Clazomenae: Fragments
and Testimonia. University of Toronto Press. Toronto.
De Pontieu, B., McIntosh, S. W., Carlsson, M., Hansteen, V. H.,
Tarbell, T. D., Schrijver, C. J., Title, A. M., Shine, R. A.,
Tsuneta, S., Katsukawa, Y., Ichimoto, K, Suematsu, Y., Shimizu,
T, Nagata, S., 2007. Chromospheric Alfvenic Waves Strong Enough
to Power the Solar Wind. Science, 318. 5856, 1574 - 1577.
Deamer, D. W. 1985. Boundary structures are formed by organic
components of the Murchison carbonaceous chondrite. Nature.
317:792-794.
Deamer, D. W., Pashley, R. M. 1989. Amphiphilic components of
the Murchison carbonaceous chondrite: surface properties and
membrane formation. Orig. Life Evol. Biophys. 19:21-38.
Doerfert1, S. N. 2009. Methanolobus zinderi sp. nov., a
methylotrophic methanogen isolated from a deep subsurface coal
seam. Int J Syst Evol Microbiol 59, 1064-1069.
Dombrowski, H. Bacteria from Paleozoic salt deposits. Annals of
the New York Academy of Sciences, 1963, 108, 453.
Dose, K., Bieger-Dose, A., Dillmann, R., Gill, M., Kerz, O.,
Meinert, H., Nawroth, T., Risi, S., Stridde, C. 1995.
ERA-experiment space biochemistry. Advances in Space Research,
16, 119-129.
Dufresne, E. R., Anders, E. 1962. On the chemical evolution of
the carbonaceous chondrites, Geochim. Cosmochim, Acta, 26,
1085-1114.
Ehrenfreund, P., Menten, K. M. 2002. From Molecular Cluds to the
Origin of Life. In G. Horneck & C. Baumstark-Khan. Astrobiology,
Springer.
Ehrenfreund, P., Glavin, D. P., Botta, O., Cooper, G., Bada, J.
2001. Extraterrestrial amino acids in Orgueil and Ivuna: Tracing
the parent body of CI type carbonaceous chondrites, PNAS 98,
2138-2141.
Ehrenfreund, P., & Sephton, M. A. (2006). Carbon molecules in
space: from astrochemistry to astrobiology. Faraday Discussions
133, 277 - 288.
Eisner, J. A., 2007, Water vapour and hydrogen in the
terrestrial-planet-forming region of a protoplanetary disk.
Nature 447, 562-564
Folk, R. L., Lynch, F. L. 1997. Nanobacteria are alive on Earth
as well as Mars [abstract], in Proceedings of SPIE The
International Society for Optical Engineering. 3111, 407-419.
Flanner, B. P., Roberage, W., & Rybicki, G. B., 1980, The
penetration of diffuse ultraviolent radiation into interstellar
clouds. The Astrophysical Journal, 236, 598-608.
Folsome, C.E., Lawless, J., Romiez, M., Ponnamperuma, C. 1971.
Heterocyclic compounds indigenous to the Murchison meteorite.
Nature 232, 108-109.
Folsome, C.E., Lawless, J., Romiez, M., Ponnamperuma, C. 1973.
Heterocyclic compounds recovered from carbonaceous chondrite.
Geochim. Cosmochim. Acta 37, 455-465.
Fredriksson, K., Kerridge, J. F. 1988. Carbonates and sulfates
in CI Chondrites: Formation by aqueous activity on the parent
body, Meteoritics, 23, 35-44.
Gerasimenko, L. M., Hoover, R. B., Rozanov, A. Yu., Zhegallo, E.
A., Zhmur, S. I. 1999. Bacterial paleontology and studies of
carbonaceous chondrites, Paleontological Journal, 33, 439-459,
1999.
Gladman, B. 2005. The Kuiper Belt and the Solar System's Comet
Disk. Science, 307, 71 - 75.
Glassgold, A. E., Najita, J., & Igea, J., 2004, Heating
protoplanetary disk atmospheres The Astrophysical Journal, 615,
972-990.
Gohn, G. S., et al., 2008. Deep Drilling into the Chesapeake Bay
Impact Structure Science, 320, 1740-1745.
Goldreich, P., Lithwick, Y., Sari, R. 2004. Final Stages of
Planet Formation. Astrophysical Journal, 614 497-507.
Gomez Y, Tafoya, D., Anglada, G., Loinard, L., Torrelles, J. M.,
Miranda, L. F., Osorio, M., Franco-Hernandez, R., Nyman, L.,
Nakashima, J., Deguchi, S. 2008. HCO+ emission possibly related
with a shielding mechanism that protects water molecules in the
young PN K 3-35. Proceedings IAU Symposium No. 251, In S. Kwok &
S. Sandford, eds. International Astronomical Union.
Greaves,, J. S. 2005. Disks Around Stars and the Growth of
Planetary Systems Science, 205, 68-71.
Greaves, J. S., Richards, A. M. S., Rice, W. K. M., Muxlow, T.
W. B. 2008. Enhanced dust emission in the HL Tau disc: a
low-mass companion in formation? Monthly Notices of the Royal
Astronomical Society: Letters 391, L74-L78.
Haisch, K. E., Lada, E. A., Lada, C. J. 2001. Disk frequencies
and lifetimes in young clusters. The Astrophysical Journal 553,
L153-L156.
Hayatsu, R., 1964. Orgueil meteorite: organic nitrogen contents.
Science 146, 1291-1293.
Hayatsu, R., Studier, M.H., Moore, L.P., Anders, E. 1975.
Purines and triazines in the Murchison meteorite. Geochim.
Cosmochim. Acta 39, 471-488.
Hayatsu, R., Studier, M., Oda, A., Fuse, K., Anders, E., 1968.
Origin of organic matter in the early solar system-II. Nitrogen
compounds. Geochim. Cosmochim. Acta 32, 175-190.
Herbst, E., Klemperer, W. 1973. The formation and depletion of
molecules in dense interstellar clouds. Astrophysical Journal,
185, 505-533.
Hester, J. J., Desch, S. J., Healy, K. R., Leshin, L. A. 2004.
The Cradle of the Solar System. Science Vol. 304. no. 5674, 1116
- 1117.
Hinrichs, K-U, et al., 2006. Biological formation of ethane and
propane in the deep marine subsurface. PNAS, 103, 14684-14689.
Hollis, J. M., Lovas, F.J., Jewell, P.R. 2000. Hydrocarbons,
polyaromatic hydrocarbons, the amino acid glycine, vinegar and
the sugar glycoaldehyde Astrophys. J. 3111, 115-136 .
Hoover, R. B. 1997. Meteorites, Microfossils, and Exobiology"
[abstract] in Instruments, Methods, and Missions for the
Investigation of Extraterrestrial Microorganisms. In Hoover, R.
B., Editor, Proceedings of SPIE Vol. 3111, 115-136.
Hoover, R.B., 1998. Meteorites, Microfossils, and Exobiology"
[abstract] in Instruments, Methods, and Missions for the
Investigation of Extraterrestrial Microorganisms. In Hoover, R.
B. Editor, Proceedings of SPIE Vol. 3111, 115-136 .
Hoover, R. B., 2006. Comets, carbonaceous meteorites, and the
origin of the biosphere. Biogeosciences Discussions, 3, 23-70.
Hoover, R. B., Rozanov, A., 2003. Microfossils, biominerals and
chemical biomarkers in Meteorites, in: Instruments Methods and
Missions for Astrobiology VI, edited by: Hoover, R. B., Rozanov,
A. Yu., and Lipps, J. H., Proc. SPIE 4939, 10-27.
Hoover, R. B., Rozanov, A. Yu., Zhmur, S. I., Gorlenko, V. M.
1998. Further evidence of micro-fossils in carbonaceous
chondrites, in: Instruments, Methods and Missions for
Astrobiology, edited by: Hoover, R. B., Proc. SPIE 3441,
203-215. 1998.
Hoover, R. B., Jerman, G., Rozanov, A. Y., Sipiera, P. B. 2004.
Indigenous microfossils in carbonaceous meteorites" [abstract].
In: Richard B. Hoover, Gilbert V. Levin and Alexei Y. Rozanov;
eds.. Proceedings of SPIE Volume 5555: Instruments, Methods, and
Missions for Astrobiology VIII, 1-17.
Horneck, G. 1993. Responses ofBacillus subtilis spores to space
environment: Results from experiments in space Origins of Life
and Evolution of Biospheres 23, 37-52.
Horneck, G., Becker, H., Reitz, G. 1994. Long-term survival of
bacterial spores in space. Advances in Space Research, Volume
14, 41-45.
Horneck, G., Eschweiler, U., Reitz, G., Wehner, J., Willimek,
R., Strauch, G 1995. Biological responses to space: results of
the experiment Exobiological Unit of ERA on EURECA I. Advances
in Space Research 16, 105-118
Horneck, G., et al., 2001. Bacterial spores survive simulated
meteorite impact Icarus 149, 285.
Horneck, G., Rettberg, P., Reitz, G., Wehner, J., Eschweiler,
U., Strauch, K., Panitz, C., Starke, V., Baumstark-Khan, C.
2001. Origins of Life and Evolution of Biospheres 31, 527-547.
Horneck, G. Mileikowsky, C., Melosh, H. J., Wilson, J. W.
Cucinotta F. A., Gladman, B. 2002. Viable Transfer of
Microorganisms in the solar system and beyond, In G. Horneck &
C. Baumstark-Khan. Astrobiology, Springer.
Hole, F. 1957. Black Cloud. Heinemann publishing, London.
Hoyle, F. and Wickramasinghe, N.C., 1978. Lifecloud: the origin
of life in the galaxy. London: J.M. Dent.
Hoyle, F., Wickramasinghe, C. 1993. Our Place in the Cosmos. J.
M. Dent, London.
Hoyle, F. Wickramasinghe, N. C. 2000. Astronomical Origins of
Life. Steps Towards Panspermia, Klewer Academic Publishers.
Imshenetsky, A.A., Lysenko, S.V. and Kazakov,G.A. (1978). Upper
boundary of the biosphere. Applied and Environmental
Microbiology, 35, 1-5.
Jacobsen, S. B., 2005. The Hf-W system and the origin of the
Earth and Moon. Annual Review of Earth and Planetary Sciences.
33, 531-570.
Jagoutz, E., Sorowka, A., Vogel, J. D., Wenke, H. 1994. ALH
84001: Alien or progenitor of the SNC family? (abstract).
Meteoritics, 29, 478-479.
Jansen, D. J., van Dishoeck, E. F., & Black, J. H. 1994.
Physical and chemical structure of the IC 63 Nebula. I.
Milimeter and far-infrared observations. Astronomy &
Astrophysics, 282, 605-620.
Joseph, R. 2000. Astrobiology, the origin of life, and the death
of Darwinism. University Press.
Joseph, R. 2008. Origins, evolution, metamorphosis, extinction
video lectures: The origin of life, myth of the Organic Soup;
life on Mars; life from meteors; Moon microbes; life in extreme
environments, extinction & evolution; the evolution of life from
other planets; the origin and creation of the sun, solar system
and life on Earth. http://Cosmology.com/Videos.html.
Joseph, R. 2009a,b. The evolution of life from other planets.
Cosmology 1, 100-200.
Joseph R. 2010. The Infinite Universe vs the Myth of the Big
Bang: Black Holes, Red Shifts, Acceleration, Life. Journal of
Cosmology, 6, In press.
Joseph R. Schild, R. 2010a. Biological Cosmology and the Origins
of Life in the Universe. Journal of Cosmology, 5, 1040-1090.
Joseph, R., Schild , R. 2010b. Origins, Evolution, and
Distribution of Life in the Cosmos: Panspermia, Genetics,
Microbes, and Viral Visitors From the Stars. Journal of
Cosmology, 7. In press.
Joseph, R., and Wickramasinghe, N. C. (2010). Comets and
contagion: Evolution and diseases from space? Journal of
Cosmology, 7, 1750-1770.
Kahn, C. H. 1994. Anaximander and the Origins of Greek
Cosmology. Hackett Publishing Company.
Kaiser, R. I., 2002. Experimental investigation on the formation
of carbon-bearing molecules in the interstellar medium via
neutral-neutral reactions. Chemical Review, 102, 1309-1358.
Kalirai, J. S., Bergeron, P., Hansen, B. M. S., Kelson, D. D.,
Reitzel, D. B., Rich, R.M., Richer, H. B. 2007. Stellar
Evolution in NGC 6791: Mass Loss on the Red Giant Branch and the
Formation of Low-Mass White Dwarfs. Astrophysical Journal 671
748-760.
Kamp. I. & Dullemond, G. P. 2004 The gas temperature in the
surface layer sof protoplanetary disks. The Astrophysical
Journal, 615, 1-24.
Kerridge, J. F. 1967. Mineralogy and genesis of the carbonaceous
meteorites, in: Mantles of the Terrestrial Planets, edited by:
Runcorn, S. K., Interscience, Wiley & Sons, New York, 35-47.
Kokubo, E. Ida, S. 2002. Formation of Protoplanet Systems and
Diversity of Planetary Systems. The Astrophysical Journal,
Volume 581, Issue 1, pp. 666-680.
Lawless, J.G., Zeitman, B., Pereira,W.E., Summons, R.E.,
Duffield, A.M. 1974. Dicarboxylic acids in the Murchison
meteorite. Nature 251, 40-42.
Lawless, J. G., Yuen, G. U. 1979. Quantification of
monocarboxylic acids in the Murchison carbonaceous meteorite.
Nature 282:396-398.
Lawless, J.G., Folsome, C.E., Kvenvolden, K.A., 1972. Organic
matter in meteorites. Sci. Am. 226, 38-46.
Liebert, J., Arnett, E., Holberg, J., Williams, K. 2005. Sirius.
Astrophysical Journal Letters. 630, L69-L72.
Liebert, K., Young, P. A., Arnett, E., Holberg, J. B., Williams,
K. A. (2005) The Age and Progenitor Mass of Sirius B. The
Astrophysical Journal Letters 630, L69-L72.
Lovett, S. T. 2006. Microbiology: Resurrecting a broken genome.
Nature 443, 517-519.
Lovis, C., Mayor, M. 2007. Planets around evolved
intermediate-mass stars. I. Two substellar companions in the
open clusters NGC 2423 and NGC 4349 Astronomy and Astrophysics
472, 657-664.
Manning, C. E., Mojzsis, S. J., Harrison, T. M. 2006. Geology.
age and origini of supracrustral rocks at Akilia, West
Greenland. American Journal of Science, 306, 303-366.
Marquis, R. E., Shin, S. Y. 2006. Mineralization and responses
of bacterial spores to heat and oxidative agents FEMS
Microbiology Reviews 14375 - 379.
Martins, Z., Botta. O., Fogel, M. L., Sephton, M. A., Glavin, D.
P., Jonathan, C., Watson, C., Dworkin, J. P., Schwartz, A. W.
2008. Pascale Ehrenfreund Extraterrestrial nucleobases in the
Murchison meteorite Earth and Planetary Science Letters 270,
130-136.
Mastrapaa, R.M.E., Glanzbergb, H ., Headc, J.N., Melosha, H.J,
Nicholsonb, W.L. 2001. Survival of bacteria exposed to extreme
acceleration: implications for panspermia, Earth and Planetary
Science Letters 189, 30 1-8.
Mayor M., Udry, S., Lovis, C., Pepe, F., Queloz, D., Benz, W.,
Bertaux, J.-L., Bouchy, F., Mordasini, C., Segransan, D. 2009.
The HARPS search for southern extra- solar planets. XIII. A
planetary system with 3 super-Earths (4.2, 6.9, and 9.2 M)
Astronomy & Astrophysics 493, 639-644.
McKay, D. S., Gibson Jr., E. K., Thomas-Keprta, K.L., Vali, H.,
Romanek, C. S., Clemett, S. J., Chillier, X.D. F., Maechling, C.
R., Zare, R. N. 1996. Search for Past Life on Mars: Possible
Relic Biogenic Activity in Martian Meteorite ALH84001. Science
273 (5277): 924-930.
McLean, R.J.C., Welsh, A.K., Casasanto, V.A., 2006. Microbial
survival in space shuttle crash. Icarus 181, 323-325.
McLean, R.J.C., McLean, M. A. C. (2010). Microbial survival
mechanisms and the interplanetary transfer of life through
space. Journal of Cosmology, 7, 1802-1820
Mimura, K., Michioki, O., Kenichiro, S., Shigemasa, H. 2007.
Selective release of D and 13C from insoluble organic matter of
the Murchison meteorite by impact shock. Meteoritics & Planetary
Science 42, 347-355.
Mitchell, F. J., & Ellis, W. L. 1971 Surveyor III: Bacterium
isolated from lunar retrieved TV camera. In AA Levinson (ed),
Proceedings of the second lunar science Conference, MIT press,
Cambridge.
Monnier, J. D., Tuthill, P. G., Lopez, B., Cruzalebes, P.,
Danchi, W. C., Haniff, C. A. 1999. The last gasps of VY Canis
Majoris: aperture synthesis and adaptive optics imagery. The
Astrophysical Journal 512, 351-361.
Montmerle, T., Augereau, J-C., Chaussidon, Marc, E. A. 10
authors (2006). Solar System Formation and Early Evolution: the
First 100 Million Years. Earth, Moon, and Planets (Spinger) 98,
39-95.
Moore, T. E., Horwitz, J. L. 1998. Thirty Years of Ionospheric
Outflow: Causes and Consequences. American Geophysical Union.
San Francisco, December.
Mojzsis, S.J., Arrhenius, G., McKeegan, K.D., Harrison, T.M.,
Nutman, A.P., Friend, C.R.L., 1996. Evidence for life on Earth
before 3,800 million years ago. Nature 384, 55-59.
Moser, D. P. et al., 2005. Desulfotomaculum and Methanobacterium
spp. Dominate a 4- to 5-Kilometer-Deep Fault. Applied and
Environmental Microbiology, 71, 8773-8783.
Nagy, B., Meinschein, W. G. Hennessy, D, J. 1961,
Mass-spectroscopic analysis of the Orgueil meteorite: evidence
for biogenic hydrocarbons. Annals of the New York Academy of
Sciences 93, 25-35.
Nagy, B., Claus, G., Hennessy, D, J., 1962, Organic Particles
embedded in Minerals in the Orgueil and Ivuna Carbonaceous
Chondrites. Nature 193, 1129 - 1133.
Nagy, B., Fredriksson, K., Kudynowkski, J., Carlson, L. 1963a,
Ultra-violet Spectra of Organized Elements. Nature 200, 565 -
566.
Nagy, B., Fredriksson, K., Urey, C., Claus, G., Anderson, C. A.,
Percy, J. 1963b. Electron Probe Microanalysis of Organized
Elements in the Orgueil Meteorite, Nature 198, 121 - 125.
Nagy, B., Bitz, M. C. 1963c. Long-chain fatty acids from Orgueil
meteorite. Archives of Biochemistry and Biophysics, 101,
240-263.
National Geographic (2008). Newborn planet is youngest ever
found National Geographic News 2008, April 3.
Nemchin, A. A., Whitehouse, M.J., Menneken, M., Geisler, T.,
Pidgeon, R.T., Wilde, S. A. 2008. A light carbon reservoir
recorded in zircon-hosted diamond from the Jack Hills. Nature
454, 92-95.
Nickerson, C.A., Ott, C.M., Wilson, J.W., Ramamurthy, R.,
Pierson, D.L., (2004). Microbial responses to microgravity and
other low-shear environments. Microbiology and Molecular Biology
Reviews 68, 345-361.
Nicholson, W. L., Munakata, N., Horneck, G., Melosh, H. J.,
Setlow, P. 2000. Resistance of Bacillus Endospores to Extreme
Terrestrial and Extraterrestrial Environments, Microbiology and
Molecular Biology Reviews 64, 548-572.
Nimmo, S.D., Hart, D.G., Korycansky, C.B. 2008. Agnor
Implications of an impact origin for the Martian hemispheric
dichotomy Nature 453 1220-1223.
Nishi, R. Nakana, T., & Umebayashi, T. 1991. Magnetic flux loss
form interstellar clouds with various grain-size distributions.
The Astrophysical Journal, 368, 181-194.
Nittler, L. R., Hoppe, P. 2005. Are Presolar Silicon Carbide
Grains from Novae Actually from Supernovae? The Astrophysical
Journal, 631, L89-L92.
Nyquist L. E., Bansal B. M., Wiesmann H., and Shih C.-Y. (1995)
"Martians" young and old: Zagami and ALH84001 (abstract). Lunar
Planet. Sci. XXVI, 1065-1066.
O'Neil, J., Carlson, R. W., Francis, E., Stevenson, R. K. 2008.
Neodymium-142 Evidence for Hadean Mafic Crust Science 321, 1828
- 1831.
Osman, S., Peeters, Z., La Duc, M.T., Mancinelli, R.,
Ehrenfreund, P., Venkateswaran, K., (2008). Effect of shadowing
on survival of bacteria under conditions simulating the Martian
atmosphere and UV radiation. Applied and Environmental
Microbiology 74, 959-970.
Pellin. M. J., Savina, M. R., Tripa. E., Calaway, W. F., Davis,
A. M., Lewis, R. S., Amari, S., Clayton, R. N. 2002. C, N, Si,
Fe, Sr, Zr, Mo AND Ba isotopic analysis of type X Murchison SiC
grain: experimental evidence for a new type of stellar
nucleosynthesis in supernova. 65th Annual Meteoritical Society
Meeting.
Pflug, H. D, 1978 Yeast-like microfossils detected in oldest
sediments of the earth Journal Naturwissenschaften 65, 121-134.
Pflug, H.D. 1984. Microvesicles in meteorites, a model of
pre-biotic evolution. Journal Naturwissenschaften, 71, 531-533.
Piaget, J. 1955. Play, Dreams and Imitation. Basic Books.
Piaget, J. 1958. The growth of Logical Thinking. Basic books.
Poitrasson, F., Alexander, N. Hallidaya, N., Leea, D-C. 2004.
Sylvain Levasseura and Nadya Teutscha, d Iron isotope
differences between Earth, Moon, Mars and Vesta as possible
records of contrasted accretion mechanisms. Earth and Planetary
Science Letters 223, 253-266.
Pillitteri, F., Favata, F., Micela, G. 2008. The X-ray
luminosity of solar-mass stars in the intermediate ageopen
cluster NGC 752. Astronomy &Astrophysics 490, 113-123.
Prasad, S. S., & Tarafdar, S. P. 1983. UV radiation field inside
dense clouds. The Astrophysical Journal, 267, 603-609.
Randel, W. J. et al., 1998. Seasonal Cycles and QBO Variations
in Stratospheric CH4 and H2O Observed in UARS HALOE Data.
Journal of the Atmospheric Sciences, 55. 163-185.
Rankenburg, K., Brandon, A. D., Neal, C. R. 2006. Neodymium
Isotope Evidence for a Chondritic Composition of the Moon
Science 312. no. 5778, 1369 - 1372.
Raymond, S. N., Quinn, T., Lunine, J. I. 2007. High-Resolution
Simulations of The Final Assembly of Earth-Like Planets. 2.
Water Delivery And Planetary Habitability Astrobiology 7, 66-84.
Rosing, M. T., 1999. C-13-depleted carbon microparticles in >
3700-Ma sea-floor sedimentary rocks from west Greenland. Science
283, 674-676.
Rosing, M. T., Frei, R., 2004. U-rich Archaean sea-floor
sediments from Greenland - indications of > 3700 Ma oxygenic
photosynthesis. Earth and Planetary Science Letters 217,
237-244.
Sahl. J. W., et al., 2008. Subsurface Microbial Diversity in
Deep-Granitic-Fracture Water in Colorado Applied and
Environmental Microbiology, 74, 143-152.
Sancho L. G., de la Torre, R., Horneck, G., Ascaso, C. , de los
Rios, A. Pintado,A., Wierzchos, J.,Schuster, M. 2007. Lichens
Survive in Space: Results from the 2005 LICHENS Experiment
Astrobiology. 7, 443-454.
Savina, M. R., Davis, A. M., Tripa, C. E., Pellin, M. J.,
Clayton, R. N., Lewis, R. S., Amari, S., Gallino, R., Lugaro, M.
2003. Barium isotopes in individual presolar silicon carbide
grains from the Murchison meteorite Geochimica et Cosmochimica
Acta, 67, 3201-3214.
Schutte, W. A., 2002, Production of organic molecules in
interstellar ices, Advances in Space Research, 30, 1409-1417.
Scheifele, L. Z., Boeke, J. D. 2008. From the shards of a
shattered genome, diversity. Proc Natl Acad Sci 105,
11593-11594.
Scientific American, September, 2009.
Schoenberg, R., Kamber, B.S., Collerson, K.D., Moorbath, S.
2002. Tungsten isotope evidence from approximately 3.8-Gyr
metamorphosed sediments for early meteorite bombardment of the
Earth. Nature 418, 403-405.
Schroder, K-P, Smith, R. C. 2008. Distant future of the Sun and
Earth revisited. Mon. Not. R. Astron. Soc. 000, 1-10.
Sephton, M.A., 2002. Organic compounds in carbonaceous
meteorites. Nat. Prod. Rep. 19, 292-311.
Sephton, M.A., Botta, O., 2005. Recognizing life in the solar
system: guidance from meteoritic organic matter. Int.
J.Astrobiology 4, 269-276.
Setlow, P. 2006. Spores of Bacillus subtilis: their resistance
to and killing by radiation, heat and chemicals. Journal of
Applied Microbiology 101, 514-525.
Setlow, B., Setlow, P. 1995. Small, acid-soluble proteins bound
to DNA protect Bacillus subtilis spores from killing by dry
heat. Appl Environ Microbiol. 61, 2787-2790.
Sharov, A.A. 2006. Genome increase as a clock for the origin and
evolution of life. Biology Direct. 1, 17.
Sharov, A.A. 2009.Exponential Increase of Genetic Complexity
Supports Extra-Terrestrial Origin of Life. Journal of Cosmology,
1, 63-65.
Snyder, L. E. 2004, The Search for interstellar glycine. Origins
of Life & Evolution of the Biosphere. 27, 115-133.
Soffen, G.A. 1965. NASA Technical Report, N65-23980.
Stochel, G., Brindell, M., Macyk, W., Stasicka, Z., &
Szacilowski, K. 2009. Bioinorganic Photochemistry. Wily. New
York.
Stoks, P.G., Schwartz, A.W., 1979. Uracil in carbonaceous
meteorites. Nature 282, 709-710.
Stoks, P.G., Schwartz, A.W., 1981. Nitrogen-heterocyclic
compounds in meteorites: significance and mechanisms of
formation. Geochim. Cosmochim. Acta 45, 563-569.
Sunde, E.P., Setlow, P., Hederstedt, L., Halle, B. 2009. The
physical state of water in bacterial spores. Proceedings of the
National Academy of Sciences of the United States of America
106, 19334-19339.
Suzuki, T. K., 2007. Evolution of Alfven wave-driven solar winds
to red giants. Proceedings of the International Astronomical
Union 3, 201-207.
Szewczyk, N.J., Mancinelli, R.L., McLamb, W., Reed, D.,
Blumberg, B.S., Conley, C.A., 2005. Caenorhabditis elegans
survives atmospheric breakup of STS-107, Space Shuttle Columbia.
Astrobiology 5, 690-705.
Thomson, W. [Lord Kelvin] 1881. Presidential Address: On the
origin of life on earth. Report of the Forty-First Meeting of
the British Association for the Advancement of Science; held at
Edinburgh in August.
Satterfield, C.L., Lowenstein, T.K., Vreeland, R.H., Rosenzweig,
W.D., Powers, D.W. 2005. New evidence for 250 Ma age of
halotolerant bacterium from aPermian salt crystal. Geology 33,
265-268.
Udry, S., Bonfils, X., Delfosse, X., Forveille, T., Mayor, M.,
Perrier, C., Bouchy, F., Lovis, C., Pepe, F., Queloz, D.,
Bertaux, J.-L. 2007. The HARPS search for southern extra-solar
planets. XI. Super-Earths (5 and 8 M{⊕}) in a 3-planet system.
Astronomy and Astrophysics 469, L43-L47.
van Dishoeck, E. F. 2006. Chemistry in low-mass protostellar and
protoplanetary regions. PNAS 103, 12249-12256.
von Helmholtz, H. 1872. in W. Thomson & P.G. Tait's Hanbuch der
Theoretishen Physik, Vol. 1, Part 2. Heildelburg.
Van der Velden, W., Schwartz, A.W. 1977. Search for purines and
pyrimidines in Murchison meteorite. Geochim. Cosmochim. Acta 41,
961-968.
Vreeland, R.H., Rosenzweig, W.D., Powers, D.W., 2000, Isolation
of a 250 million-year-old halotolerant bacterium from a primary
salt crystal. Nature, 407, 897-900.
Wachter, A., Winters, J. M., Schroder, K.-P., Sedlmayr, E. 2008.
Dust-driven winds and mass loss of C-rich AGB stars with
subsolar metallicities Astronomy & Astrophysics manuscript no.
wwss2008 c ESO 2008 May 23, 2008, 1-9.
Wadhwa, M., Lugmair G. W. 1996. The formation age of carbonates
in ALH 84001 (abstract). Meteoritics, 31, A145.
Wainwright, M., Fawaz Alshammari, F., Alabri, K. (2010). Are
microbes currently arriving to Earth from space? Journal of
Cosmology, 2010, Vol 7, 1692-1702.
Werner, M., Ott, U., Begemann, F. 1994. Measurements on silicon
carbide from Murchison meteorite: Data for C, N, and SI
Meteoritics 29, 550-551.
Woese, C. 1968. The Genetic Code. Harper & Row.
Wilson, J.W., et al., (2007). Space flight alters bacterial gene
expression and virulence and reveals a role for global regulator
Hfq. Proceedings of the National Academy of Sciences of the
United States of America 104, 16299-16304.
Wittkowski, M., Langer, N., Weigelt, G. 1998.
Diffraction-limited speckle-masking interferometry of the red
supergiant VY CMa". Astronomy and Astrophysics (European
Southern Observatory) 340: 39-42.
Yamamato, T. 1985. Formation environment of cometary nuclei in
the primordial solar nebula. Astronomy & Astrophysics, 142,
31-36.
Yuen, G., Blair, N., Des Marais, D.J., Chang, S., 1984. Carbon
isotope composition of low molecular weight hydrocarbons and
monocarboxylic acids from Murchison meteorite. Nature 307,
252-254.
Yurimoto, H., & Kuramoto, K. 2004. Molecular cloud origin for
the oxygen isotope heterogeneity in the solar system, Science,
305, 1763-1766.
Zhmur, S. I., Gerasimenko, L. M. 1999. Biomorphic forms in
carbonaceous meteorite Alliende and possible ecological system -
producer of organic matter hondrites" in Instruments, Methods
and Missions for Astrobiology II, RB. Hoover, Editor,
Proceedings of SPIE Vol. 3755 p. 48-58.
Zhmur, S. I., Rozanov, A. Yu., Gorlenko, V. M. 1997. Lithified
remnants of microorganisms in carbonaceous chondrites,
Geochemistry International, 35, 58-60.
|