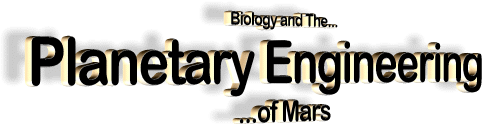
by
Julian A. Hiscox
Department of Microbiology
BBRB 17, Room 361
University of Alabama at Birmingham
Birmingham, Al 35294-2170, USA.
from
TheCaseForMars Website
I. Introduction
From the perspective of biology, planetary engineering is the
ability to alter the environment of a planet so that terrestrial
organisms can survive and grow (McKay, 1982).
The feasibility of
altering planetary environments is clearly demonstrated by mankind's
activities on the Earth (Levine, 1991; Fogg, 1995a) and it is
increasingly apparent that in the near term future mankind will gain
the technological capability to engineer the climate of Mars.
Current thought experiments/proposals for the planetary engineering
of Mars differ in their methodology, technical requirements,
practicality, goals and environmental impact (reviewed and discussed
by Fogg, 1995b).
The planetary engineering of Mars may be divided into two distinct
mechanistic steps, ecopoiesis followed by terraforming.
Ecopoiesis,
a term derived by Haynes (1990) which, when applied to Mars, can be
viewed as the creation of a self-regulating anaerobic biosphere. On
the other hand, terraforming refers to the creation of a human
habitable climate (discussed in Fogg 1995b).
Whether the creation of
such biospheres are possible is not known (Fogg, 1989; Pollack and
Sagan, 1993; Fogg, 1995b). However, the majority of these planetary
engineering models invoke the use of biological organisms, both
during alteration of the planetary environment and in the regulation
of the resulting biosphere.
This article will briefly review the
implications of the current Martian environment and assets for
biology and then discuss the relationship between biology and
planetary engineering.
II. Current
Martian environment and implications for biology
At present the Martian surface environment is effectively
sterilizing for all forms of terrestrial organisms (Rothschild,
1990; Mancinelli and Banin, 1995; Dose et al. 1995), although some
protected niches may exist above and below the surface of Mars (Friedmann,
1986; Thomas and Schimel, 1991; Boston et al. 1992; Rothschild,
1990, 1995).
The properties of the Martian environment that would
preclude the survival and growth of terrestrial organisms are as
follows (but see also McKay (1982); Rothschild (1990); Banin and
Mancinelli, (1995); Mancinelli and Banin (1995)):
1. Low pressure
The atmospheric pressure on Mars (Table 1), mostly
due to carbon dioxide, varies from approximately 7.4 to 10 millibar
(mbar) (Hess et al. 1980). Extremely low pressure damages organisms
and can affect efficient DNA repair (Ito, 1991; Koike et al. 1991).
2. Low temperature
The average diurnal temperature ranges from
approximately 170 K to 268 K. During the Martian summer the
temperature perhaps rises above the freezing point of water at some
equatorial latitudes.
From temperature requirements alone, organisms
would not be able to survive on present day Mars for a number of
reasons:
-
First, the temperatures would completely freeze any
organism and depending on the freezing process would cause cellular
damage through the formation of ice crystals.
-
Second, such low
temperatures would raise the activation energy for enzyme catalyzed
processes and thus inhibit biochemical/metabolic reactions.
-
Third,
biochemical reactions occur in solution and the transport of
metabolites would not occur efficiently in a ice crystals.
3. Water
Liquid water which is a prerequisite for life (McKay,
1991; McKay and Stoker, 1989), under the current Martian atmospheric
pressure is unstable. Such extreme dry conditions would cause
dehydration, for example damaging DNA (Dose et al. 1995) and leading
to mutation and cell/organism death.
4. Radiation
The main source of radiation at the Martian surface is
ultraviolet (UV) radiation between the wavelengths of 190 and 300
nm.
UV-radiation can be lethal. It is absorbed by nucleic acids
(i.e. DNA) and activates the chemical formation of various adjuncts
that inhibit replication and transcription of DNA. In the absence of
an ozone layer, organisms can only escape the lethal affects of
UV-radiation by living in protected habitats.
Even those surface
organisms which have efficient DNA and cellular repair enzymes would
probably perish.
5. Oxidants
Due to the continuous bombardment of the Martian
surface with UV-radiation the topmost layer of the regolith is
thought to contain strong oxidants which are damaging for cellular
components.
6. Carbon dioxide
As mentioned previously the major atmospheric
component is carbon dioxide (Table 1).
In organisms the relatively
high concentration of carbon dioxide would probably cause a low
intracellular pH. i.e. acidosis which may be damaging for cellular
proteins, cellular components and metabolism (Hiscox and Thomas,
1995).
7. No organic
material
Because of the continuous bombardment of
UV-radiation and oxidizing conditions, no organic material will be
present on the Martian surface (Bullock et al. 1994 and references
there in).
Table 1
Mars-atmospheric composition and partial
pressure of the most abundant gases.
(Data from Fogg 1995c, Hiscox 1995
and references therein).
Species |
Abundance by Volume |
Partial Pressure |
CO2 |
0.9532 |
7 mbar |
N2 |
0.027 |
0.2 mbar |
Ar |
0.016 |
minor |
O2 |
0.0013 |
minor |
CO |
0.0007 |
minor |
H2O |
0.0003 |
minor |
Ne |
2.5 ppm |
very minor |
Kr |
0.3 ppm |
very minor |
Xe |
0.8 ppm |
very minor |
O3 |
0.04 to 0.2 ppm |
extremely minor |
III. Biologically
useful Martian resources
Undoubtedly the current Martian environment is extremely hostile for
terrestrial life.
However, Mars does contain sufficient volatiles to
enable some form of colonization and perhaps planetary engineering
to render environmental conditions more clement for terrestrial life
to survive and grow (Meyer and McKay, 1984, 1989; McKay et al.
1991a; Fogg, 1995c; Zubrin, 1995).
Analysis of Martian soil and shergottites, nakhlites and chassignittes (SNC) meteorites (believed
to have been ejected from Mars - Mustard and Sunshine, 1995 and
references therein) has shown that all of the elements necessary
for carbon based life on Earth are present on Mars (Dreibus and
Wanke, 1987; Gooding, 1992; Banin and Mancinelli, 1995).
It is evident that Mars once possessed a more clement climate and
many observable surface features have been attributed to the
presence of liquid water and a dense carbon dioxide atmosphere
(Carr, 1986; 1987).
Many planetary engineering scenarios (see Fogg,
1995c and references there in) propose that it may be possible to
return Mars to an earlier such climate using planetary engineering
techniques (with the proviso that such volatiles are still present).
Fogg (1995c) suggests that unless impact erosion (Melosh and
Vickery, 1989) "blasted" the atmosphere into space then huge
quantities of volatiles are still likely to reside on the planet.
Over geological history Mars may have lost more volatiles than it
gained. For example, water may also have been lost by hydrodynamic
escape, atmospheric spluttering and other mechanisms (refer to Carr,
1987; Jakosky, 1991; Kass and Yung, 1995). Therefore returning Mars
to a past climatic state may not be possible, and clearly given the
climatic history of Mars such a climate maybe geologically unstable
and undesirable for the extreme long term habitability of the
planet.
A number of compounds and elements are absolutely required for life;
liquid water, the so called CHNOPS (carbon, hydrogen, nitrogen,
oxygen, phosphorous and sulfur) are the main elements which
constitute amino acids (which make up proteins) and nucleotides
(which make up DNA and RNA) and various minerals are also required.
All of these elements/compounds are believed to be present on Mars (Banin
and Mancinelli, 1995). The amount and location of these resources on
Mars is briefly reviewed below.
For a more in depth reviews refer to Fogg (1995b,c); Meyer and McKay, 1989, 1991a; and Banin and
Mancincelli (1995).
1. Water
Currently, the surface of Mars is
devoid of liquid water and the atmosphere only contains minute
amounts of water vapor (Table 1)(Carr, 1987). The two main
sources of remaining water on Mars are thought to be the north
polar cap and the
regolith. The quantity of water on Mars is
uncertain, and estimates range in order of magnitudes,
equivalent to a layer of water over the planet 13 meters (m) to
100 m (Squyres and Carr, 1986).
The north polar cap is composed mainly of water ice (Kieffer et
al. 1976). The equatorial regions of Mars appear to be ice poor
whereas the heavily cratered terrain pole-ward of ± 30° latitude
appears to be ice rich (Squyres and Carr, 1986), with perhaps a
conservative estimate of the equivalent of 17 m of ice spread
over the surface of Mars (Jankowski and Squyres, 1993).
How much
liquid water would be necessary, or indeed liberated by either ecopoiesis and/or terraforming has not been determined. However,
based on current data, a detailed model for the hydrological
cycle on Mars has been proposed (Clifford, 1993) and perhaps
this could be adapted for modeling the hydrological cycle during
ecopoiesis/terraforming.
Mars will probably never be a wet planet as it might have been
in the past (Carr, 1986; 1987), although the view that Mars was
"warm and wet" is uncertain and perhaps "cold and icy" may be
more appropriate (Kasting, 1991; Squyres and Kasting, 1994).
However, there will probably be sufficient water for some type
of a biosphere to be established.
For certain, the water
requirement for ecopoiesis will be several orders of magnitude
less than that for a terraformed biosphere. Ultimately, it may
be possible to import water onto Mars, for example by the
redirection of ice asteroids into the Martian atmosphere to
release their volatile components (see Fogg, 1995b).
However,
although such proposition might be technically feasible, the
number of asteroids needed to be diverted is very large.
2. Buried organic material
Bullock et al. (1994) estimate that
organic material, either deposited by meteorites and/or remains
from an earlier biosphere, maybe between 3 and 40 meters from
the surface or perhaps be present in polar regions (Bada and
McDonald, 1995).
These deposits could therefore be utilized by
plants that have long root systems and/or by subsurface
microorganisms.
However, such scenarios depend on how long it
would take thermal waves to penetrate through the ground during
planetary engineering.
3. Carbon
On first inspection the two main
sources of "trapped" carbon dioxide are as a solid in the polar
caps and adsorbed in the regolith. These sources are thought to
exchange between 10 and 100 times the current atmospheric
pressure of CO2 via the atmosphere and are thus thought to
regulate climate change on Mars (Fanale et al. 1982).
The
permanent cap at the south pole is thought to contain at the
most around 10 mbar of CO2 (Fanale and Cannon, 1979) (however
this figure is uncertain). Due to the uncertainty in the extent
of the Martian regolith, the total mineral surface area exposed
to the Martian atmosphere is not known.
However, laboratory
simulations of the simultaneous adsorption of H2O and CO2 (Zent
and Quinn, 1995), where palagonite is used as an analogue of the
Martian regolith (Zent et al. 1987), would appear to confirm
that the current absorbed inventory of CO2 is 30-40 mbar.
An even greater source of CO2 may be combined in the form of
carbonate. Carbonates would have been formed by CO2, present in
the early Martian atmosphere, dissolving in water and combining
with cations such as Ca2+, Fe2+ and Mg2+ and subsequent
precipitates forming carbonates (refer to McKay and Nedell, 1988
and references there in).
Warren (1987) suggests that the
regolith's low Ca/Si ratio is due to the fact that Ca was
removed from the regolith as calcium carbonate. Warren (1987)
estimates that perhaps a global shell 20m thick would suffice to
remove 1000 mbar of CO2 from the Martian atmosphere. Whether
this amount of carbonate is present is not known.
However, the
layered deposits observed in the Valles Marineris (Nedell et al.
1987) (believed to be an ancient water system) are thought to be
derived from the precipitation of 30 mbar of atmospheric CO2 as
carbonate in lakes (McKay and Nedell, 1988).
4. Nitrogen
One of the main limiting factors for
the growth of "Martian" organisms could be the low abundance of
nitrogen (Table 1). No direct analysis of the nitrogen content
on the surface of Mars has yet been conducted, the proportion of
nitrogen in the Martian atmosphere is shown in Table 1.
The
abundance of nitrogen on the surface of Mars has been estimated
from analysis of SNC data (for example Grady et al. 1995) and it
would appear that there is proportionally less nitrogen on Mars
than on the Earth (Banin and Mancinelli, 1995).
Therefore, from
the planetary engineer's perspective it is crucial that forth
coming Mars missions investigate the abundance (and perhaps
distribution) of nitrogen containing compounds.
5. Minerals
Minerals are also essential for
biological process, for example as co-factors in enzyme
catalyzed reactions and components of vitamins. All of the
elements necessary to support terrestrial life are thought to be
present on Mars, although as with the CHNOPS elements their
concentration compared to Earth are either slightly higher,
lower or the same (Banin and Mancinelli, 1995).
Mineral deposits, carbonates and nitrates etc. may be located in
ancient evaporate basins (Forsythe and Zimbelman, 1995) and
given suitable locations, i.e. at equatorial latitudes (maximum
surface temperature), low point (maximum atmospheric pressure),
these may be ideal areas for establishing pioneer ecosystems.
Indeed, locations where ancient Martian life may have flourished
would contain subsurface organics that have been buried
sufficiently deep enough to be protected from oxidation (Zent
and McKay, 1994).
However, as mentioned above, depending on
their depth, these deposits may remain in deep freeze and thus
inaccessible for a long periods of time. Locations for ancient
Martian life include old oceans along northern planes (Helfer,
1990), ancient ice-covered lakes (Scott et al. 1991; Andersen et
al. 1995) and evaporites (Rothschild, 1990).
Therefore, site
selection to establish these ecosystems may closely resemble
site selection for Martian exobiology (Rothschild, 1990; Farmer
et al. 1995).
IV. Initial planetary
engineering-a biological perspective
For Mars to be less hostile for pioneer organisms initial planetary
engineering will be required to increase the atmospheric pressure.
This will have a number of effects, including an increase in surface
temperature, liquid water will be stable (at least at equatorial
latitudes) and an increase in ozone abundance that will reduce the
amount of UV-radiation reaching the surface.
Perhaps the simplest
way to do this, as discussed below, will be to liberate CO2
deposits using a runaway greenhouse mechanism.
1. Runaway greenhouse mechanisms and
greenhouse gases
To initiate the runaway greenhouse
mechanism for warming Mars, an initial warming is required to
release CO2, this will act as a greenhouse gas increasing the
global temperature leading to the release of more CO2 and so on
(Haynes, 1990; McKay et al. 1991b; Zubrin and McKay, 1993).
A
number of mechanisms have been proposed to provide this initial
warming step. Two techniques being orbiting mirrors to reflect
sunlight onto polar regions acting alone or in conjunction with
the in situ production of the greenhouse gases such as
chlorofluorocarbons (CFCs) (McKay et al. 1991b; Zubrin and
McKay, 1993).
Estimates of the lifetime of CFCs in the Martian atmosphere vary
from a few days (Levine, 1991-quoted in Fogg, 1992) to 100 years
(Zubrin and McKay, 1993). Therefore, if the half-life of CFCs in
the Martian atmosphere is small, the production of such
quantities of CFCs to warm Mars may be impractical (Fogg, 1992).
The Levine estimate of CFC lifetimes maybe an under estimate as
this was based on a current Martian environment in which the O3
layer is very small and thus more UV-radiation is available to
degrade the CFCs. If solar mirrors could be used to produce an
increase in the pCO2 then a greater ozone layer would form (via
the photo-dissociation of CO2) thus increasing the lifetime of
the CFCs.
However, as Fogg (1992) points out, such CFCs may not
co-exist with an ozone layer in a planetary engineered
atmosphere, as the photo-dissociation products of CFCs are
thought to react with O3 and therefore reduce ozone coverage.
As
discussed below, ozone will be important in reducing the amount
of UV radiation on the surface of Mars so that terrestrial
organisms may exist unprotected on the surface. Instead of using
CFCs as a greenhouse gas it maybe possible to use alternative
greenhouse agents such as
perfluorocarbons (see Fogg, 1995b).
However, the toxicity of perfluorocarbons at the concentrations
required for warming Mars would have to be determined.
An alternative greenhouse gas for warming Mars could be ammonia
(NH3) (Pollack and Sagan, 1991). Ammonia rich asteroids could be
diverted towards the Martian atmosphere to release their
quantity of NH3 (Pollack and Sagan, 1991; Zubrin and McKay,
1993). However, the probability of locating asteroids that are
composed of 100% NH3 is unlikely.
The composition of any comet
is unlikely to contain more than 10% NH3, therefore the problem
is again a matter of scale. Also, NH3 has been shown to be very photochemically unstable in primitive terrestrial atmospheres
(which may resemble Martian planetary engineered environments)
and NH3 life times are estimated to be from 10 (Kasting, 1982)
to 40 years (Kuhn and Atreya, 1979).
Therefore the economic cost
of importing NH3 containing asteroids might be more than the in
situ production of some type of halocarbon to produce an
equivalent greenhouse warming. However, as discussed in section
six, there maybe a biological solution to this problem.
At a conservative estimate, perhaps only 500 mbar of CO2 is
available for release using the runaway greenhouse mechanisms.
Based on the work of Kasting (1989; 1991), this would result in
a surface warming of approximately 240 K, perhaps bringing
temperatures at the equator (during the Martian summer) above
the freezing point of water.
(Note: Kasting (1989) is based upon
a model of the climate of early Earth and assumes a 0.8-bar N2
background atmosphere and a 30% reduction in stellar luminosity-
the insulation on Mars is approximately 50% that of Earth).
Pollack (1991) estimates that CO2 pressures on the order of
several bars were required to raise the annually averaged
temperature at low latitudes on an early Mars to values in
excess of 273 K and this is also in agreement with the
calculations of McKay et al. (1991b) for planetary engineering.
Thus using the runaway greenhouse mechanisms of planetary
engineering, the climate of Mars would probably be cold and icy
rather than warm and wet.
2. Nanotechnology
Alternatively, in concert with the
previous techniques or alone,
nanotechnology may be employed for
planetary engineering (Morgan, 1994; Nussinov et al. 1994) .
For
example in the liberation of carbon dioxide from carbonate
deposits (Nussinov et al. 1994). Great claims are made to the
potential exponential growth of nano-robots (Freitas, 1983;
Morgan, 1994). Morgan (1994) has suggested that nano-robots
could contain structures similar to those found in biological
organisms. In common with microorganisms, nano-robots may have a
huge growth capacity, i.e. doubling time, which for some
bacteria, growing under ideal conditions, can be as little as 20
minutes. Ideal growth conditions for nano-robots are therefore
likely to resemble those found for microorganisms (see Figure
1.).
However, conditions on Mars will not be ideal for grow of
either microorganisms or nano-robots. Nutrients/substrates may
vary in abundance, there may be competition for resources etc.
Therefore, growth is likely to be linear rather than exponential
(Figure 1).
Also, unlike biotechnology, nanotechnology has not
been demonstrated.
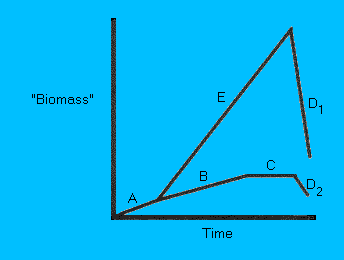
Figure 1.
Growth curves of "organisms" (either microorganisms or nano-robots) on Mars. (A) Is the lag phase in which the
"organisms" are growing at a slow rate. In microorganisms this
caused by the "turn on" of genes to make new proteins etc. If
conditions are optimal, i.e. abundant substrate/nutrients, and
remain optimal, then growth rate becomes exponential (E).
However, if ecological climax is reached, e.g. the substrate
pool becomes limiting, then the population crashes (D1). A far
more likely scenario is that the initial number of "organisms"
grows slowly (B) as the distribution of substrates will not be
uniform.
Eventually, the number of organisms "living" will equal
the number of organisms "dying" (C). If the substrate becomes
limiting or environmental conditions worsen (i.e. drop in
temperature) then the number of organisms will drop (D2). As
conditions become more favourable then growth resumes (A).
For
Mars, the ideal growth curve for any organism should follow (A
to C or D2). This idea of keeping growth rates below climax has
been rightly argued by Fogg (1995b).
3. Nuclear mining and alternative
planetary engineering mechanisms
There are a number of mechanisms
available for liberating the carbon dioxide "trapped" as
carbonates, including cometary impact (Fogg, 1989 and references
there in) and nuclear mining (Fogg, 1989; 1992; Pollack and
Sagan, 1991).
Such anthropogenic mechanisms of planetary
engineering become attractive if there is insufficient volatile
inventory for a runaway greenhouse mechanism. The environmental
consequences of radioactive fall out associated with certain
forms of nuclear mining could be quite severe (Haynes and McKay,
1992), leading perhaps to widespread mutation and death of
organisms.
Given an advanced technology (more than that required
for ecopoiesis) it may be possible to release carbon dioxide in
carbonate deposits by volcanic means. The thermal erosion of
carbonates has been hypothesised as a mechanism for the
recycling of carbon dioxide into the atmosphere of early Mars
(Schaefer, 1993).
4. Ozone
One of the main functions of initial
planetary engineering would be to increase the ozone layer thus
providing shielding of organisms from UV-radiation (Hiscox and
Lindner, 1996).
Based on O3 estimates in a Precambrian
atmosphere, the minimum ozone column being tolerable by
unprotected bacteria would fall between 1x1018 and 4x1018 cm2
depending on the bacterial species being considered (Francois
and Gerard, 1988). Fortuitously, oxygen is not required to
generate an ozone layer, instead the photodissociation of CO2
might be used to generate sufficient ozone to provide an ozone
layer (Hiscox and Lindner, 1996).
Such a scenario may be
self-regulating (Figure 2).
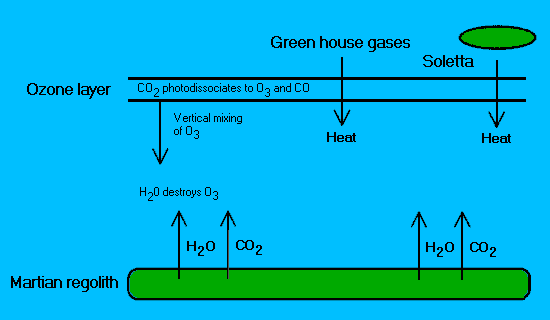
Figure 2.
Diagrammatic representation of an ozone "cycle" during
planetary engineering. (Interactions at the poles are complex
and thus for simplicity are not represented).
Ozone is created
by the photodissociation of carbon dioxide. Through vertical
mixing this reaches the lower atmosphere where it is destroyed
by water, which has been released from the regolith by heating
either with solettas (Birch, 1992) and/or greenhouse gases
(McKay et al. 1991b).
(Note: the hypothetical greenhouse gases
used in this scenario do not chemically react with ozone. More
carbon dioxide is released leading to the formation of new ozone
and so on.)
If only a minimum ozone coverage is created by planetary
engineering (sufficient to provide shielding against lethal
UV-radiation for most organisms), on some occasions the ozone
level may drop below a threshold level.
Thus exposed organisms
may be exposed to lethal levels of UV-radiation on Mars.
Seasonal and latitudinal variations in dust and cloud opacities
have induced as much as a 40% variation in ozone on a seasonal
and latitudinal basis (Lindner, 1988). In addition, the
asymmetry in dust and cloud opacities at late winter in each
hemisphere could also cause a 10-20% hemispherical asymmetry in
ozone (Lindner, 1988). Therefore a mechanism of preventing this
drop in ozone would be preferable.
The current dust
concentration in the Martian atmosphere can induce a 10-50%
increase in ozone abundances because photodissociation rates are
greatly reduced by dust absorption (Lindner, 1988) and this
phenomena has been observed in the polar regions of Mars, where
dust absorbs or scatters to space most UV-radiation before it
strikes the cap (Lindner, 1990).
Therefore a planetary engineering mechanism that can create such
a dust storm would be useful in providing additional protection
to organisms by reducing the amount of UV-radiation reaching the
surface. First by providing direct shielding against
UV-radiation and second by inducing localised increases in the
production of ozone, thus restoring an ozone layer.
One
mechanism to generate a global dust storm may be heating of the
polar regions with space based sunlight reflectors (Zubrin and
McKay, 1993) (abbreviated to SBR). Similar to what occurs on
Mars at the moment, the asymmetric heating of one pole would
cause a pressure differential i.e. wind, and this would carry
dust.
However, if the polar reserves of carbon dioxide and water
are liberated early in planetary engineering then an alternative
mechanism is required. Such a mechanism could be the heating of
a near by dusty area on Mars by a SBR (Hiscox and Lindner,
1996). This may cause a localised dust storm which would provide
local UV-radiation coverage by plugging the nearby ozone hole.
Satellites could be used to monitor atmospheric ozone abundances
and warn of impending ozone "holes".
5. Temperature/humidity
Different microbial species vary
widely in their optimal temperatures for growth. The upper end
of temperature range tolerated by any given species correlates
well with the general thermal stability of that species'
proteins. Microorganisms share with plants and animals the heat
shock response, a transient synthesis of a set of "heat shock
proteins" when exposed to a sudden rise in temperature above the
growth optimum.
These proteins appear to be unusually heat
resistant and act to stabilise the heat sensitive proteins of
the cell. However, beyond a certain temperature proteins will
irreversibly denature and therefore enzymes (which are mostly
composed of proteins) will become non-functional. Some bacteria
can also exhibit cold shock, the killing of cells by rapid as
opposed to slow cooling.
For example, rapid cooling of
Escherichia coli from 310 to 278 K will kill 90% of the cells.
Early stages of planetary engineering will probably require psychrophilic forms, i.e. those that grow best at low
temperatures (normally 288-293 K).
In order to define a minimum temperature and humidity for
pioneer microorganisms to grow during ecopoiesis one can study
microorganisms that inhabit regions on the Earth that best
approximate regions on Mars. Apart from the greater pressure and
less UV-radiation, the cold dry Ross Desert regions of
Antarctica best approximate Mars (Friedmann and Weed, 1987;
McKay, 1993).
Yet these regions are host to a variety of
microorganisms which live just under the surface of rocks and
these are called endolithic microorganisms (Friedmann, 1982). In
these regions air temperatures range between 258 K and 273 K in
the summer and may drop to near 213 K in the winter, with
relative humidities ranging from 16 to 75 percent (Friedmann,
1982 and references there in).
Before planetary engineering,
Mars will be colder than Antarctica, however, as discussed
above, using the greenhouse mechanism it may be possible to
raise the surface temperature of Mars to conditions resembling Antartica.
Microbial activity in the Antarctic cryptoendolithic habitat is
regulated by temperature (Nienow et al. 1988a) and metabolic
activity is possible only when solar radiation raises the
temperature of the rock above 263 K (Nienow et al. 1988b).
Therefore the minimum Martian surface temperature required for
ecopoiesis, should 263 K or greater (at least in regions were
organisms will be seeded).
Cryptoendolithic lichens begin photosynthesis when the matric
water potential is -46.4 megaPascals (MPa) which corresponds to
a relative humidity of 70% at 281 K, whereas cryptoendolithic
cyanobacteria photosynthesize at high matric water potentials of
-6.9 (and greater) (a relative humidity of 90% at 281 K) (Palmer
Jr. and Friedmann, 1990).
Alternatively, both may use melt-water
as a source of water rather than water vapour which is used in
times of environmental stress. Therefore, if melt water is
unavailable for pioneer microorganisms, the relative humidity
should be at least 70%, perhaps lower if genetic engineering
(see below) can be used to increase tolerance to desiccation.
Alternatively, pioneer microorganisms could be adapted to
tolerate desication (Friedmann, 1995-personal communication in
Hiscox and Thomas, 1995), and this is perhaps a more feasible
mechanism than genetic engineering.
6. Growth and diversity
After the introduction of
microorganisms into a partially altered Martian environment the
growth rate will exceed the death rate and therefore there
should be a net accumulation of microorganisms.
However, once
the new biosphere becomes established the population of
microorganisms in a stable biosphere will be roughly constant,
i.e. growth is balanced by death.
The survival of any microbial
group within its niche is determined in large part by successful
competition for nutrients and by maintenance of a pool of living
cells (or dormant cells) during nutritional deprivation. In a
constantly changing environment, as will occur during planetary
engineering, the proportion of living bacteria to dead bacteria
may vary dramatically (Figure 1).
V. Candidate
biological methods and mechanisms for adapting terrestrial organisms
to grow on Mars
A number of pioneer microorganisms and plants have been proposed for
introduction onto a partially altered Mars (Averner and MacElroy,
1976; Friedmann and Ocampo-Friedmann, 1994; Hiscox, 1995; Hiscox and
Thomas, 1995; Fogg, 1995d).
The first organisms will of necessity be
photoautotrophic (Haynes and McKay, 1992), which means that they utilise sunlight as an energy source and do not require complex
organic material for metabolism (which would be absent on the
surface of the planet prior to the introduction of terrestrial
microorganisms-see section two).
In order to aid organisms to
survive and more importantly grow as soon as physically possibly on
a partially altered Mars, two main mechanisms of adaptation can be utilised either individually or in concert, that of genetic
manipulation and/or directed selection under simulated Martian
conditions (Hiscox, 1995; Hiscox and Thomas, 1995) (Figure 3):
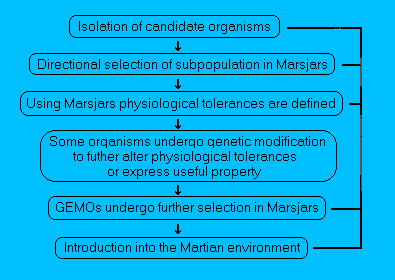
Figure 3.
Schematic representation of selecting organisms for growth
on Mars.
Candidate organisms could perhaps be isolated from extremes
of environments on the Earth that in some respects resemble the
partially altered environment on Mars. The organisms could be
further adapted to Mars by either genetic engineering and/or
selection in Marsjars.
Once environmental conditions become more
clement on Mars, organisms could be directly introduced from the
Earth with minimum adaptation.
(The stage at which organisms could
be introduced onto Mars is indicated by the right-hand path - Taken
from Hiscox, 1996).
1. Genetic engineering
Genetic engineering is now common place and
the ability to manipulate organisms for Mars, especially prokaryotes
and also eukaryotes is entirely feasible (Hiscox, 1995).
For
example, a pioneer microorganisms's tolerance to lower intracellular
pH could be increased by engineering in a gene(s) from another
organism that confers tolerance to low pH (Hiscox and Thomas, 1995).
Such an organism would then be termed recombinant, or in this case a
genetically engineered Mars organism (GEMO; Hiscox, 1995).
One
danger in introducing new genes into an organism is that the over
expression of such a gene may lead to deficiencies in other key
metabolites, therefore the inter-conversion of biosynthetic
components has to be tightly regulated (Hiscox, 1995; Hiscox and
Thomas, 1995).
2. Genetic selection
Alternatively, organisms could be adapted for
growth on a partially altered Mars by growing them under simulated
environmental conditions that increasingly resembles the climate on
Mars at the proposed time of their introduction. In genetic terms,
this process is called directed selection and is a well known
Darwinian concept.
In which adaptation results from the systematic
relationships between genotype and phenotype and between phenotype
and reproductive success in a given environment. There are limits to
increases in both physiological and metabolic processes using
selection, and thus genetic engineering could be used to increase
some of these. Because of their fairly rapid generation time,
microorganisms would best lead themselves to this type of
adaptation.
A number of studies have grown various terrestrial microorganisms
under different combinations of Martian or extreme
terrestrial/non-terrestrial environmental conditions (for example
see: Ito, 1991; Koike et al. 1991; Moll and Vestal, 1992) and the
growth on Mars of a blue-green algae has been modelled (Kuhn et al.
1979).
It is certainly feasible to conduct Marsjar simulations using
terrestrial microorganisms and such experiments would provide data
for the growth of terrestrial organisms in Martian greenhouses and
planetary protection issues. Indeed many of these types of
experiments have already been proposed for planetary protection
issues (Lindberg and Horneck, 1994).
The only factor of a Martian
environment that would be difficult to simulate is the effect of
gravity.
A fine balance between survival and evolutionary potential has to be
struck by organisms that have the efficient ability to remove most
errors in DNA replication. In general, an organism with perfect
replication will never evolve, although genetic recombination (gene
swapping) may still occur and act as a mechanism for evolution (and
is perhaps the major driving force!). Whereas an organism with a
highly error-prone mechanism would not survive.
The error repair
mechanism in bacteria is so accurate that an error is generated only
once in 108 to 109 bases (a base is a unit of a chromosome). Because
the genomes of bacteria are about 4.5 million bases long, only about
1% of the progeny have alterations in their base sequence. This
error level can be easily tolerated, it also continuously generates
variants that can be selected under specialized conditions. One must
bear in mind that selection is always for survival, a given species
has no advantage in evolving into a different species.
Natural
selection tends to promote the divergence of populations living in
different environments. Radical changes in the habitat, as will
occur during planetary engineering, will often exterminate a
species, therefore organisms will have to be able to adapt to these
changing circumstances.
It is increasingly evident that many microorganisms exist in
consortia formed by representatives of different genera. Other
microorganisms often characterized as single cells in the laboratory
form cohesive colonies in the natural environment.
This property of
organisms will be important during Marsjar simulations and
subsequent introduction onto Mars.
3. Safety issues of genetic engineering
Almost certainly GEMOs/selected
organisms will be released on the surface of Mars, either through
contamination associated with manned exploration, colonist's
greenhouses or the deliberate release during a planetary engineering
effort.
These organisms will be growing under conditions that do not
occur on the Earth, and therefore their evolution may proceed in a
completely novel manner compared to their counterparts on the Earth
(Haynes, 1990).
For example, non- pathogenic bacteria may become
pathogenic. Such considerations are especially important if terraforming is
realized and the human population will inhabit the
surface of Mars, although many genetic safeguards can be built into
such organisms (Hiscox, 1995).
VI. Uses of
terrestrial organisms on Mars
Terrestrial organisms will serve a number of purposes, both during
and after planetary engineering:
1. Increase in atmospheric pressure and change in chemical
composition
For example, microorganisms could be used to release
carbon dioxide from carbonate deposits (Friedmann et al. 1993) and
nitrogen from nitrate deposits (Thomas, 1995; Hiscox and Thomas,
1995) and appropriate deposits could be determined from orbit (Hiscox,
1995).
In order to terraform Mars, McKay (1982) and McKay et al.
(1991b) proposed that plants could be used to convert the mainly
carbon dioxide atmosphere formed during ecopoiesis into an oxygen
atmosphere.
For example, Fogg (1992) estimates that 5.7x1017 kg of
biomass would have to be sequestered as part of the biological
production of 158 mbar of oxygen. Also, Fogg (1995d) has addressed
some of the issues and suggests a number of solutions for growing
plants in low oxygen concentrations that would be present during
early stages of ecopoiesis i.e. below an oxygen pressure of 20 mbar.
It should be noted that previous estimates of the time taken to
convert a mainly carbon dioxide atmosphere into an oxygen atmosphere
may be underestimates as these calculations did not take into
account the possible increase in respiring aerobic organisms (i.e.
lichen, bacteria etc.) that may concomitantly increase in numbers
with more oxygen availability and result in the production of more
carbon dioxide.
Therefore, biology on Mars must be actively held
away from ecological climax in order to maximize oxygen production
and minimize its uptake (Fogg, 1995e).
One should note that if plants are to be used to convert the mainly
carbon dioxide atmosphere into an atmosphere suitable for human
habitation, then in the early stages of this process all such plants
should be either self or wind pollinating. Self pollination would
probably be the preferred option as wind pollination may be
extremely inefficient if the population density of plants is too
low.
These two mechanisms of pollination are required because the
carbon dioxide atmosphere will be too toxic for insects that
pollinate plants.
2. Climate regulation and control
Organisms will help maintain the
gaseous composition of the Martian atmosphere and thus regulate
climate.
After planetary engineering, organisms such as plants will
also affect climate by cycling vast amounts of water. An example is
provided by Amazonia, which contains two-thirds of all above ground
freshwater on Earth.
At least half of Amazonia's moisture is
retained within the forest ecosystem, being constantly transpired by
plants before being precipitated back into the forest, with a mean
cycling time of 5.5 days (Salati and Nobre, 1992).
3. Control of albedo
Sagan (1973; 1980) proposed that plant growth
could be used to lower the albedo of the Martian polar caps thus
increasing their absorption of solar radiation and heating them,
thus hopefully triggering a runaway greenhouse effect. (This
scenario has one main problem in that metabolic reactions do not
occur at the temperatures found on the Martian polar caps).
However,
the idea does have great merit for stabilizing the albedo on Mars.
For example Amazonia and Zaire forests stabilize the albedo on Earth
(Gash and Shuttleworth, 1992).
4. Replace biogeochemical cycles
The Earth's biotas are pumps for
the major bio-geochemical cycles (Schlesinger, 1991).
From a longer
term perspective, because Mars is believed to lack tectonic activity
and therefore organisms such as microbes (Thomas, 1995) and plants (Fogg,
1995d) may play an essential role in the regulation of global
nitrogen, carbon and other mineral cycles (McKay, 1982; Fogg; 1993;
Thomas 1995).
Whether purely biological cycles could replace
bio-geochemical ones is a large problem facing "biological"
planetary engineering (McKay, 1982; Fogg, 1995b; Thomas, 1995).
5. Hydrological functions
Plants play a part in hydrological cycles
in addition to those discussed in (i), by controlling water runoff.
Vegetation permits a slower and more regulated run-off, allowing
water supplies to make a steadier and more substantive contribution
to their ecosystems, instead of quickly running off into streams and
rivers- possibly resulting in flood and drought regimes downstream.
As the hydrosphere is gradually activated on Mars so these
hydrological cycle becomes more important.
It will be important to
ensure that water is cycled by transpiration and rainfall.
6. Production of greenhouse gases
Microorganisms could be used to metabolise nitrate deposits to NH3. As discussed in section four,
NH3 is a powerful greenhouse gas, so not only would this process
contribute to the warming of the planet, but at low levels NH3 would
be photochemically broken down into N2, a further greenhouse gas
(H2O) and H2 (Kasting, 1982).
(However, this pathway maybe
undesirable as the H2 produced would probably be lost to space (Fox,
1993 and references therein).
Another green house gas that could be
produced by biological mechanisms is methane, CH4. Methane may have
been a constituent of the Martian paleoatmosphere (Kasting, 1991).
However, methane is rapidly photodissociated by UV-radiation, but an
increase in ozone and efficient/abundant production of CH4 by
biological organisms may partially mitigate this problem and lead to
a net accumulation of CH4.
7. Biomass production and soil protection
On early Earth reduced
organic material formed by fixation of carbon dioxide and carbonates
was ultimately utilized by other organisms scouring the debris of
destroyed cells.
Thus pioneer microorganisms and subsequent
generations will provide a pyramid of biomass for successive
generations of organisms. (During initial planetary engineering the
Martian surface will rarely be refreshed by rainfall and will be
unable to retain moisture. Therefore hardy microorganisms which were
able to utilize water vapor could be used to build up a "top
soil").
The spread and settlement of vegetation protects soil cover. On
Earth soil erosion is a major problem in many areas of the world,
for example, it leads to declines in soil fertility.
Although no
soil is present on Mars with the growth of appropriate
microorganisms gradually a biomass will begin to build up and the
planting of trees, grasses and long rooted plants could, as on
Earth, could be used to prevent large scale erosion (Figure 4).
8. Production of materials for colonists
Provided the relevant
organisms can grow on Mars, these would include trees to provide
wood for construction, food and medicines, antibiotics from fungi
etc.
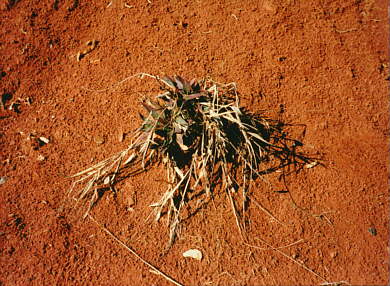
Figure 4.
Photograph of plants on Mars. Once the oxygen level is
around 20 mbar then plants can be introduced onto Mars. These will
serve a number of functions including the production of more oxygen
and stabilizing geological features.
A drainage channel caused by
the recent flow of water can be observed in the background. In the
foreground plants are growing and spreading toward the drainage
channel preventing further erosion.
(Photograph J. A. Hiscox and M.
W. Parnell).
VII. The
importance of biodiversity in planetary engineering
Also a key question is how many species are required to establish a
stable ecosystem, either leading to Vitanova or Terranova?
This
concept is known as biodiversity and encompasses all life forms from
the planetary species to populations of species together with their
ecosystems and ecological processes.
On Earth biodiversity plays two
critical roles.
-
Biodiversity provides the biosphere with a
medium for energy and material flows, which in turn provide
ecosystems with their functional properties.
-
It supports and
creates ecosystem resilience, which will be absolutely crucial on
Mars. Resilience can be defined as the ability of ecosystems to
resist stresses and shocks, to absorb disturbance and to recover
from disruptive change.
All of these processes will be occurring
during planetary engineering and indeed occur on Earth.
The concept
can be expressed more formally, it connotes an equilibrium-theory
idea to the effect that ecosystems with their cybernetic mechanisms
display homeostatic attributes that allow them to maintain function
in the face of stress induced structural changes (Cairns and Pratt,
1995).
Biodiversity will be important during and after planetary
engineering on Mars, one useful definition is of
environmental/ecosystems services which reflect environmental
functions and ecological processes and can be defined as any
functional attributes of natural ecosystems that are demonstrably
beneficial to mankind (Cairns and Pratt, 1995).
Although, it is
difficult to speculate on the composition of Martian ecosystems and
to draw extrapolate from terrestrial ecosystems, on Earth the values
provided by such systems include generating and maintaining soils,
converting solar energy into plant tissue, sustaining hydrological
cycles, running bio-geochemical cycles (including the elements
carbon, nitrogen, phosphorus and sulphur), controlling the gaseous
mixture of the atmosphere (which helps to determine climate - i.e.
through the CO2/H2O greenhouse effect) and regulating weather and
climate at both macro and micro-levels.
Thus they basically include
three forms of processing, namely of minerals, energy and water (Perrings,
1987).
Ecological services at first inspection often depend to appear not
so much on biodiversity but on biomass. For example, when a patch of
forest is replaced by a monoculture, the new vegetation can supply
certain of the same ecological functions (and perhaps more
efficiently), including photosynthesis, protection of soil cover,
atmospheric processing and hydrological functions.
However, on
closer inspection biodiversity is extremely important, a monoculture
may provide less cycling of nutrients and other soil nutrients and
be more prone to disease.
VIII.
Ramifications for the Martian environment of planetary engineering
During planetary engineering geological features will change, for
example if the global temperature raises above 273 K then water in
the form of ice will gradually begin to melt in the regolith.
This
has a number of consequences, for example, if rivers begin to form,
the associated erosion may bring to the surface any buried organic
material. Another important point to emphasize is that biology on
Mars, at least during the initial stages of planetary engineering
must always be used to add CO2/O2/N2 /greenhouse gases to the
atmosphere.
It would be undesirable to reach a point where
microorganisms initiate a global freezing because all of the CO2 has
been re-sequestered as organic carbon.
The introduction of terrestrial microorganisms into the Martian
environment, whether in greenhouses or for planetary engineering
will obviously affect the search for any extinct, but especially
extant Martian life.
Before planetary engineering commences and
during the initial stages the very surface of Mars will be
sterilizing for all forms of terrestrial life, whether genetically
modified/adapted or not. However, if oasis of life do exist, then
such enclaves may be over run by terrestrial organisms. Or perhaps
if environmental conditions become more clement during planetary
engineering such organisms will compete with terrestrial organisms.
Therefore, a thorough search for "life" on Mars will perhaps be
necessary before the wide spread introduction of terrestrial
organisms.
IX. The dynamics of
Martian environmental change versus the capabilities of a biological
engine
For the "biological engine" to facilitate any planetary engineering
effort certain environmental conditions discussed in section two
will have to modified by non-biological means before organisms can
be introduced. Most importantly a decrease in UV radiation and an
increase in surface temperature above the freezing point of water.
As discussed in section four, these conditions could both be
accomplished by an increase in the atmospheric pressure. Undoubtedly
the biological engine is very powerful, witness the conversion of
the anaerobic environment on the early Earth to an aerobic biosphere
via photosynthesis, a biological mechanism.
Although, as Thomas
(1995) points out, concrete data in the area of the biological
engine is lacking and comparisons with terrestrial equivalents may
be difficult to draw. Such predictions as to the effectiveness of a
biological engine on Mars are hampered by four main factors; the
composition, state and distribution of the volatile inventory and
the performance of organisms under Martian conditions (Haynes,
1990).
The forth coming Mars Pathfinder and Surveyor missions may
provide some answers to the former three points and Marsjar
simulations to the later.
X.
Colonists/greenhouses and planetary engineering
Colonists and planetary engineering are very interrelated. The
presence of colonists on the Martian surface has been proposed to be
the main driving force behind the ultimate terraforming of Mars (Fogg,
1993).
However, colonists and colonies on Mars will provide an
integral role in assessing the feasibility of a planetary
engineering scenario in a number of ways:
1. Simulating biological systems and planetary engineering in
greenhouses
In order to become less dependent on supplies from
Earth, such colonies are likely to utilize greenhouses for a number
of purposes including food production and waste
processing/recycling.
Such greenhouses could be viewed as giant Marsjars as the atmosphere inside the vessels might, in part,
resemble the atmosphere at some point during planetary engineering,
such as the Terrariums proposed by the Obayashi Corporation
(Ishikawa et al. 1990; 1993).
For example, the spread of organisms
throughout the Martian soil, biomass production and plant growth
e.g. respiration versus photosynthesis in a high CO2 environment
could be simulated and modeled.
The composition of a planetary engineered atmosphere has not been
modeled in detail and colonist's greenhouses would probably contain
more water than would be liberated by near term planetary
engineering scenarios. One point to note is that H2O2 release by the
Martian "top soil" may be toxic for organisms in the greenhouse (Zent
and McKay, 1994).
To overcome this problem efficient venting may be
used, at least until the H2O2 production decreases to more tolerable
levels. Alternatively, deeper soil deposits that do not contain
oxides (Bullock et al. 1995) could be used.
2. Detailed volatile inventory
Colonists/explorers will be best
able to assess the volatile inventory and distribution of materials
essential for planetary engineering on Mars (Haynes, 1990; Haynes
and McKay, 1992; Fogg, 1995c) and Antarctic research outposts may
provide a useful model for this process (Andersen et al. 1990).
XI. From
Vitanova to Terranova
Almost certainly, given near term technology, some form of
ecopoiesis can be accomplished on Mars and Haynes (1990) suggested
such a planet may be named Vitanova.
Terraforming is more dependent
on sufficient volatile inventory and is thus more uncertain than
ecopoiesis. However, if terraforming is possible, i.e. to create
Terranova (Haynes, 1990), then one of the main biological problems
to be faced may be the environmental change from an anaerobic to an
aerobic biosphere.
On the early Earth a stepwise improvement in anaerobic metabolism
allowed cells to survive and multiply wherever they could find
simple nutrients in solution. A similar process may occur during
ecopoiesis. However, after several billion years on the early Earth,
the accumulation of free oxygen in the atmosphere brought about a
radical change in the biosphere. The anaerobes retreated to
unaerated environments and newly evolved aerobes took over the
surface.
Bacteria that could survive the toxic effects of oxygen
could also capitalize on the more efficient metabolism it supported.
This luxury may not be afforded to organisms that have prospered
during ecopoiesis. McKay et al. (1991b) calculated an oxygen
biosphere may be obtained in 21,000 to 100,000 years via
photosynthesis.
This is considerably less time than the switch from
an anaerobic to an aerobic biosphere in the history of the Earth.
Therefore, anaerobic organisms may perish and ecosystems and the
biosphere disrupted. The remains of these organisms may provide
biomass for the organisms that remain or those that are to come.
However, the consequences and benefits of such a decision to proceed
with terraforming Vitanova must be carefully weighed with the risk
of failure (Haynes, 1990).
XII.
Conclusions
In conclusion, in full agreement with McKay (1982), Haynes (1990)
and Fogg (1995d) the relationship between biology and the planetary
engineering of Mars can only be more accurately investigated when
the volatile inventory, chemical state and geological distribution
is determined.
Also, extensive analysis of the performance of GEMOs
and terrestrial microorganisms using Marsjars will be required.
However, given the suitable abundance of such volatiles and moderate
advances in technology, there is no biological reason why the goal
of at least Vitanova cannot be realized.
Acknowledgments
I wish to extend my thanks to the following people for providing
both valuable discussions, suggestions and advice:
-
Martyn Fogg
-
Imre
Friedmann
-
Bob Haynes
-
Lee Lindner
-
Chris McKay
-
Tom Meyer
References
1. Andersen, D. T., C. P. McKay, R.
A. Wharton Jr. and J. D. Rummel. 1990. An Antarctic research
outpost as a model for planetary exploration. Journal of the
British Interplanetary Society 43, 499-504.
2. Andersen, D. T., P. Doran, D. Bolshiyanov, J. Rice, V.
Galchenko, N. Cherych, R. A. Wharton, C.P. McKay, M. Meyer and
V. Garshnek. 1995. A preliminary comparison of two perennially
ice-covered lakes in Antarctica: Analogs of past Martian
lacustrine environments. Advances in Space Research 15, 199-202.
3. Averner, M, M. and R. D. MacElroy. 1976. On the habitability
of Mars: An approach to planetary ecosynthesis. NASA SP-414.
4. Bada, J. L. and G. D. McDonald. 1995. Amino acid racemization
on Mars: Implications for the preservation of biomolecules from
an extinct Martian biota. Icarus 114, 139-143.
5. Banin, A. and R. L. Mancinelli. 1995. Life on Mars? 1. The
chemical environment. Advances in Space Research 15, 163-170.
6. Birch, P. 1992. Terraforming Mars quickly. Journal of the
British Interplanetary Society 45, 331-340.
7. Boston, P. J., M. V. Ivanov and C. P. McKay. 1992. On the
possibility of chemosynthetic ecosystems in subsurface habitats
on Mars. Icarus 95, 300-308.
8. Bullock, M. A., C. R. Stoker, C. P. McKay and A. P. Zent.
1994. A coupled-soil-atmosphere model of H2O2 on Mars. Icarus
107, 142-154.
9. Cairns, J. and J. R. Pratt. 1995. 63-76. In Evaluating and
monitoring the health of large-scale ecosystems. (Eds D. J.
Rapport, C. L. Gaudet and P. Calow. Springer, Berlin.
10. Carr, M. H. 1986. Mars: A water rich planet? Icarus 68,
1887-216.
11. Carr, M. H. 1987. Water on Mars. Nature 326, 30-35.
12. Clifford, S. M. 1993. A model for the hydrologic and
climatic behaviosr of water on Mars. Journal of Geophysical
Research 98, 10973-11016.
13. Dose, K., C. Stridde, R. Dillmann, S. Risi and A. Bieger-Dose.
1995. Biochemical constraints for survival under Martian
conditions. Advances in Space Research 15, 203-210.
14. Dreibus, G. and H. Wanke. 1987. Volatiles on Earth and Mars:
A comparison. Icarus 71, 225-240.
15. Fanale, F. P., and W. A. Cannon. 1979. Mars carbon dioxide
adsorption and capillary condensation on clays: Significance for
volatile storage and atmospheric history. Journal of Geophysical
Research 84, 8404.
16. Fanale, F. P., J. R. Salvail, W. B. Banerdt and R. S.
Saunders. 1982. Mars: The regolith-atmosphere-cap system and
climate change. Icarus 50, 381-407.
17. Farmer, J., D. Des Marais, R. Greeley, R. Landheim and H.
Klien. 1995. Site selection for Mars exobiology. Advances in
Space Research 15, 157-162.
18. Fogg, M. J. 1989. The creation of an artificial dense
Martian atmosphere: A major obstacle to the terraforming of
Mars. Journal of the British Interplanetary Society 42, 577-582.
19. Fogg, M. J. 1992. A synergic approach to terraforming Mars.
Journal of the British Interplanetary Society 45, 577-582.
20. Fogg, M. J. 1993. Dynamics of a terraformed Martian
biosphere. Journal of the British Interplanetary Society 46,
293-304.
21. Fogg, M. J. 1995a. Terraforming: A review for
environmentalists. The Terraforming Report 2, No.2., 92-111.
22. Fogg, M. J. 1995b. Terraforming: Engineering Planetary
Environments. SAE International, Warrendale, PA.
23. Fogg, M. J. 1995c. Exploration of the future habitability of
Mars. Journal of the British Interplanetary Society 48, 301-310.
24. Fogg, M. J. 1995d. Terraforming Mars: Conceptual solutions
to the problem of plant growth in low concentrations of oxygen.
Journal of the British Interplanetary Society 48, 427-434.
25. Fogg, M. J. 1995e. Personal communication.
26. Forsythe, R. D., and J. R. Zimbelman. 1995. A case for
ancient evaporite basins on Mars. Journal of Geophysical
Research 100, 5553-5563.
27. Fox, J. L. 1993. On the escape of oxygen and hydrogen from
Mars. Geophysical Research Letters 20, 1847-1850.
28. Francois, L. M., and J. C. Gerard. 1988. Ozone, climate and
biospheric environment in the ancient oxygen-poor atmosphere.
Planetary Space Science 36, 1391-1414.
29. Friedmann, E. I. 1982. Endolithic microorganisms in the cold
Antarctic desert. Science 215, 1045-1053.
30. Friedmann, E. I. 1986. The Antarctic cold desert and the
search for traces of life on Mars. Advances in Space Research 6,
265-268.
31. Friedmann, E. I. and R. Weed. 1987. Microbial trace-fossil
formation, biogenous, and abiotic weathering in the Antarctic
cold desert. Science 236, 703-705.
32. Friedmann, E. I. and R. Ocampo-Friedmann. 1994. A primitive
cyanobacterium as pioneer microorganism for terraforming Mars.
Advances in Space Research 15, 243-246.
33. Friedmann, E. I., M. Hua and R. Ocampo-Friedmann. 1993.
Terraforming Mars: Dissolution of carbonate rocks by
cyanobacterium. Journal of the British Interplanetary Society
46, 291-292.
34. Frietas, R. A. 1983. Terraforming Mars and Venus using
self-replicating machines. Journal of the British Interplanetary
Society 36, 139-142.
35. Gash, J. H. C. and W. J. Shuttleworth. 1992. 123-124. In
Tropical forests and climate. (Ed. N. Myers). Kluwer Academic,
Dordrecht, The Netherlands.
36. Gooding, J. L. 1992. Soil mineralogy and chemistry on Mars:
Possible clues from salts and clays in SNC meteorites. Icarus
99, 28-41.
37. Grady, M. M., I. P. Wright and C. T. Pillinger. 1995. A
search for nitrates in Martian meteorites. Journal of
Geophysical Research 100, 5449-5455.
38. Haynes, R. H. 1990. Ethics and planetary engineering. 1.
Ecce ecopoiesis: Playing God on Mars. In D. Macniven (Ed). Moral
Expertise, 161-183. Routledge, London and New York.
39. Haynes, R. H., and C. P. McKay. 1992. The implantation of
life on Mars: Feasibility and motivation. Advances in Space
Research 12, 133-140.
40. Helfer, H. L. 1990. Of Martian atmospheres, oceans and
fossils. Icarus 87, 228-235.
41. Hess, S. L., J. A. Ryan, J. E. Tillman, R. M. Henry and C.
B. Leovy. 1980. The annual cycle of pressure on Mars measured by
Viking Landers 1 and 2. Geophysical Research Letters 7, 197-200.
42. Hiscox, J. A. 1995. Modification of microorganisms for Mars.
The Terraforming Report 2, 136-150.
43. Hiscox, J. A. 1996. Planetary engineering: The Science of
genesis. Science Spectra. In press.
44. Hiscox, J. A. and D. J. Thomas. 1995. Modification and
selection of microorganisms for growth on Mars. Journal of the
British Interplanetary Society 48, 419-426.
45. Hiscox, J. A. and B. L. Lindner. 1996. Ozone considerations
and the habitability of Mars. Manuscript in preparation.
46. Ishikawa, Y., T. Ohkita and Y. Amemiya. 1990. Mars
habitation 2057. Concept design of a Mars settlement in the year
2057. Journal of the British Interplanetary Society 43, 505-512.
47. Ishikawa, Y., O. Gwynne, Y. Yamamoto, and H. Uyeda. 1993.
Construction method and procedure for early-phase Mars base. The
Case for Mars V.
48. Ito, T. 1991. The effects of vacuum-UV radiation (50-190 nm)
on microorganisms and DNA. Advances in Space Research 12,
249-253.
49. Jakosky, B. M. 1991. Mars volatile evolution: Evidence from
stable isotopes. Icarus 94, 14-31.
50. Jankowski, D. G. and S. E. Squryes. 1993. "Softened" Impact
craters on Mars: Implications for ground ice and the structure
of the Martian megaregolith. Icarus 106, 365-379.
51. Kass, D. M. and Y. L. Yung. 1995. Loss of atmosphere from
Mars due to solar wind-induced spluttering. Science 268,
697-699.
52. Kasting, J. F. 1982. Stability of ammonia in the primitive
terrestrial atmosphere. Journal of Geophysical Research 87,
3091-3098.
53. Kasting, J. F. 1989. Long-term stability of the Earth's
climate. Palaeogeography, Palaeoclimatology, Palaeoecology 75,
83-95.
54. Kasting, J. F. 1991. CO2 condensation and the climate of
early Mars. Icarus 94, 1-13.
55. Kieffer, H. H., S. C. Chase, T. Z. Martin, E. D. Minor and
F. D. Palluconi. 1976. Martian north pole summer temperatures:
Dirty water ice. Science 194, 1341.
56. Koike, J., T. Oshima, K. A. Koike, H. Taguchi, R. Tanaka, K.
Nishimura and M. Miyaji. 1991. Survival rates of some
terrestrial microorganisms under simulated space conditions.
Advances in Space Research 12, 271-274.
57. Kuhn, W. R. and S. K. Atreya. 1979. Ammonia photolysis and
the greenhouse effect in the primordial atmosphere of the Earth.
Icarus 37, 207-213.
58. Kuhn, W. R., S. R. Rogers and R. D. MacElroy. 1979. The
response of selected terrestrial organisms to the Martian
environment. Icarus 37, 336-346.
59. Levine, J. S. 1991. Terraforming Earth and Mars. Mars: Past,
present, and future: Proceedings of the Conference, Wiliamsburg,
VA, 17-26.
60. Lindberg, C. and G. Horneck. 1994. Planetary protection
considerations for Marsnet and Mars sample return missions.
Advances in Space Research 15, 277-280.
61. Lindner, B. L. 1988. Ozone on Mars: The effects of clouds an
airborne dust. Planetary Space Science 36, 125-144.
62. Lindner, B. L. 1990. The Martian polar cap: Radiative
effects of ozone, clouds and airborne dust. Journal of
Geophysical Research 95, 1367-1379.
63. Mancinelli, R. L. and A. Banin. 1995. Life on Mars? 2.
Physical restrictions. Advances in Space Research 15, 171-176.
64. McKay, C. P. 1982. Terraforming Mars. Journal of the British
Interplanetary Society 35, 427-433.
65. McKay, C. P. 1991. Urey prize lecture: planetary evolution
and the origin of life. Icarus 91, 93-100.
66. McKay, C. P. 1993. Relevance of Antarctic microbial
ecosystems to exobiology. Antarctic Microbiology, 593-601.
Wiley-Liss Inc.
67. McKay, C. P. and S. S. Nedell. 1988. Are there carbonate
deposits in the Valles Marineris, Mars? Icarus 73, 142-148.
68. McKay, C. P. and C. Stoker. 1989. The early environment and
its evolution on Mars: Implications for life. Reviews of
Geophysics 27, 189-214.
69. McKay, C. P., T. R. Meyer, P. J. Boston, M. Nelson, T.
Maccallum and O. Gwynne. 1991a. Resources of near-Earth space. (Eds
J. Lewis, M. Matthews and M. L. Guerrieri). University of
Arizona Press.
70. McKay, C. P., O. B. Toon and J. F. Kasting. 1991b. Making
Mars habitable. Nature 352, 489-496.
71. Melosh, H. J. and A. M. Vickery. 1989. Impact erosion of the
primordial atmosphere of Mars. Science 338, 487-489.
72. Meyer, T. R. and C. P. McKay. 1984. The atmosphere of
Mars-resources for the exploration and settlement of Mars. The
Case for Mars. (Ed. P. J. Boston). American Astronautical
Society Science and Technology Series 57, 209-232.
73. Meyer, T. R. and C. P. McKay. 1989. The resources of Mars
for human settlement. Journal of the British Interplanetary
Society 42, 147-160.
74. Moll, D. M. and J. R. Vestal. 1992. Survival of
microorganisms in smectitie clays: Implications for Martian
exobiology. Icarus 98, 233-239.
75. Morgan, C. R. 1994. Terraforming with nanotechnology.
Journal of the British Interplanetary Society 47, 311-318.
76. Mustard, J. F. and J. M. Sunshine. 1995. Seeing through the
dust. Martian crustal heterogeneity and links to the SNC
meteorites. Science 267, 1623-1625.
77. Nedell, S. S., S. W. Squyres and D. W. Andersen. 1987.
Origin and evolution of the layered deposits in the Valles
Marineris, Mars. Icarus 70, 409-441.
78. Nienow, J. A., C. P. McKay and E. I. Friedmann. 1988a. The
cryptoendolithic microbial environment in the Ross Desert of
Antarctica: Light in the photosynthetically active region.
Microbial Ecology 16, 271-2289.
79. Nienow, J. A., C. P. McKay and E. I. Friedmann. 1988b. The
cryptoendolithic microbial environment in the Ross Desert of
Antarctica: Mathematical models of the thermal regime. Microbial
Ecology 16, 253-270.
80. Nussinov, M. D., S. V. Lysenko and V. V. Patrikeev. 1994.
Terraforming of Mars through terrestrial microorganisms and
nanotechnological devices. Journal of the British Interplanetary
Society 47, 319-320.
81. Palmer, Jr., R. J. and E. I. Friedmann. 1990. Water
relations and photosynthesis in the cryptoendolithic microbial
habitat of hot and cold deserts. Microbial Ecology 19, 111-118.
82. Perrings, C. 1987. Economy and environment: A theoretical
essay on the interdependence of economic and environmental
systems. Cambridge University Press, UK
83. Pollack, J. B. 1991. Kuiper Prize Lecture: present and past
climates of the terrestrial planets. Icarus 91, 173-198.
84. Pollack, J. B., and C. Sagan. 1991. Planetary Engineering. (Eds
J. Lewis, M. Matthews and M. L. Guerrieri). In Resources of Near
Earth Space. University of Arizona Press.
85. Rothschild, L. J. 1990. Earth analogs for Martian life.
Microbes in evaporites, a new model system for life on Mars.
Icarus 88, 246.
86. Rothschild, L. J. 1995. A "cryptic" microbial mat: A new
model ecosystem for extant life on Mars. Advances in Space
Research 15, 223-228.
87.
Sagan, C. 1973. Planetary engineering on Mars. Icarus 20,
513-514.
88. Sagan, C. 1980. Cosmos. Random House, New York.
89. Salati, E. and C. A. Nobre. 1992. 177-196. In Tropical
forests and climate. Ed. N. Myers. Kluwer Academic, Dordrecht,
The Netherlands.
90. Schaefer, M. W. 1993. Volcanic recycling of carbonates on
Mars. Geophysical Research Letters 20, 827-830.
91. Schlesinger, W. H. 1991. Biogeochemistry: An analysis of
global change. Academic Press, San Diego.
92. Scot, D. H., J. W. Rice, Jr. and J. M. Dohm. 1991. Martian
paleolakes and waterways: Exobiological implications. Origins of
Life and Evolution of the Biosphere 21, 189-198.
93. Squyres, S. W. and M. H. Carr. 1986. Geomorphic evidence for
the distribution of ground ice on Mars. Science 231, 249-252.
94. Squyres, S. W. and J. F. Kasting. 1994. Early Mars: How warm
and how wet? Science 265, 744-749.
95. Thomas, D. J. 1995. Biological aspects of the ecopoiesis and
terraforming of Mars: Current perspectives and research. Journal
of the British Interplanetary Society 48, 415-418.
96. Thomas, D. J. and J. P. Schimel. 1991. Mars after the Viking
missions: Is life still possible? Icarus 91, 199-206.
97. Warren, P. H. 1987. Mars regolith versus SNC meteorites:
Possible evidence for abundant crustal carbonates. Icarus 70,
153-161.
98. Zent, A. P. and C. P. McKay. 1994. The chemical reactivity
of the Martian soil and implications for future missions. Icarus
108, 146-157.
99. Zent, A. P. and R. C. Quinn. 1995. Simultaneous adsorption
of carbon dioxide and water under Mars-like conditions and
application to the evolution of the Martian climate. Journal of
Geophysical Research 100, 5341-5349.
100. Zent, A. P., F. P. Fanale and S. E. Postawko. 1987. Carbon
dioxide: Adsorption on palagonite and partitioning in the
Martian regolith. Icarus 71, 241-249.
101. Zubrin, R. 1995. The economic viability of Mars
colonisation. Journal of the British Interplanetary Society 48,
407-414.
102. Zubrin, R. M. and C. P. McKay. 1993. Technological
requirements for terraforming Mars. AIAA 93-2005. 1-14, 29th
Joint Propulsion Conference and Exhibit.
|