
by James B. Pollack and Carl Sagan
1993
NASA Ames Research Center - Cornell University
from
NationalSpaceSociety Website
Abstract
Assuming commercial fusion power, heavy lift vehicles and major
advances in genetic engineering, we survey possible late-21st
century methods of working major transformations in planetary
environments.
Much more Earth-like temperatures may be produced,
-
on
Mars by generating low freezing-point greenhouse gases (e.g., CO2,
NH3, CFCs) from indigenous materials or by transporting them from
elsewhere
-
on Venus by cancelling the greenhouse effect with
high-altitude absorbing fine particles, or by a sunshield at the
first Lagrangian point, and/or by sequestering or transforming CO2
at the surface
-
on Titan by greenhouse and/or fusion warming
To
produce global environments suitable for plants and animals,
including humans, requires modifying the atmospheric composition and
mass and altering the surface temperatures on these bodies.
In
general, engineering congenial worlds for plants is much easier than
for humans, and is also a useful means of working further
modification of the atmospheric composition, especially the
establishment of several hundred mbar of O2 from H2O.
Establishing
global habitats suitable for humans will require the addition of at
least several hundred mbar of N2 and O2 into the Martian atmosphere;
the removal of most of the CO2 in Venus' atmosphere (most plausibly
by forming carbonate minerals) plus the addition of large amounts of
water; and the addition of several hundred mbar of O2 to Titan's
atmosphere.
Climatologically active abundances of some gases may be
toxic to humans. It is not clear that any of these schemes are
technically feasible (much less cost effective) with technologies
projected for the end of the next century.
They also raise
disturbing questions about environmental ethics. Global warming on
Earth has already led to calls for mitigation by planetary
engineering - e.g., emplacement and replenishment of reflective or
anti-greenhouse layers at high altitudes, or sun-shields in space.
But here especially we must be concerned about precision, stability,
and inadvertent side-effects.
The safest and most cost-effective
means of countering global warming of the Earth - beyond, e.g.,
improved energy efficiency, CFC bans and alternative energy sources
- is the continuing reforestation of ~2.5 x 107 km2
of the Earth's
surface.
This can be accomplished with present technology.
I. GENERAL
PRINCIPLES
Human technology is now able to affect the terrestrial atmosphere
and climate
on a global scale.
Three mechanisms have drawn particular attention
in recent years:
-
Global warming through a side-effect of modern technology - the
increasing greenhouse effect. It arises chiefly from the burning of
fossil fuels (releasing CO2), from the industrial production of
chlorofluorocarbons (releasing CFC s), and from cattle and rice
paddies (which release CH4).
Global warming of several K over the
next century might, it is feared, lead to drought, desertification
in mid-latitude continental interiors, massive agricultural failure
and relocation, a global rise in sea level and the flooding of
coastal cities and island nations.
(see, e.g., Hansen et al. 1988;
Strong 1989; MacCracken et al. 1990).
-
Ozonosphere depletion. It is thought to arise chiefly from the
industrial production of chlorofluorocarbons (CFC s) and threatens -
through a significant increase in the surface flux of solar
near-ultraviolet radiation (WMO 1985; NASA 1988) - not just
increases in skin cancer and a weakening of the human immune system,
but an assault on the primary photosynthetic producers at the base
of the food chain.
-
Nuclear winter. This is a long-overlooked climatic consequence of
nuclear war.
Even 1% of the 1992 global strategic arsenals - if
targeted on city centers and, especially, petroleum refineries and
storage depots - seems capable of reducing Northern Hemisphere
temperatures by as much as 10 to 20 K in continental interiors in a
time scale of the order of 10 days (and then relax back to
unperturbed conditions on a time scale of months).
The principal
mechanism by which nuclear winter works is by undoing the greenhouse
effect ("anti-greenhouse effect"), which happens when appreciable
sunlight is absorbed above the greenhouse gases.
(Turco et al.
1983,1990; Pittock et al. 1986; Sagan and Turco 1990).
Among the
predicted consequences of nuclear winter following a full nuclear
exchange in Northern spring or summer is the devastation of Northern
Hemisphere agriculture and the subsequent deaths by starvation of
vast numbers of people.
(Harwell and Hutchinson 1985).
These three processes demonstrate the general proposition that
humans can now alter environments on a planetary scale.
This
conclusion follows even if we were to entertain serious reservations
about the canonical values of the speed or severity of these effects
within the range of uncertainty in current atmospheric modeling.
There may be mitigating effects that have not yet been identified
and that just counterbalance each of these catastrophes, but such
effects have certainly not been demonstrated, and hoping for such a
deus ex machina may be tantamount to exercising what psychiatrists
call denial.
In none of these three examples is a carefully designed stable
change brought about; instead, the changes are haphazard and
inadvertent, and almost always constitute an unpleasant surprise to
those responsible for the relevant technology.
It seems possible,
therefore, if present trends continue, that within the
not-too-distant future human technology should be capable of even
more major alterations, both intentional and inadvertent. An
important issue is whether any can cause improvements rather than
deterioration in the planetary environment - perhaps with
high-precision negative feedbacks.
After the discovery of the inclement high temperatures at the
surface of Venus, it was natural to try to imagine a possible
technological fix to make that planet more Earth-like (Sagan 1961).
A wide variety of schemes have been suggested in the subsequent
scientific literature, usually to transform Venus or Mars into a
more Earth-like environment (see, e.g., Avemer and MacElroy 1976;
Burns and Harwit 1973; Fogg 1987,1989; Oberg 1981).
This subject was
earlier described as "terraforming," a term coined by the science
fiction writer Jack Williamson under the pseudonym Will Stewart in a
series of stories published in the 1940's.
However, it is possible
to imagine a range of environmental alterations other than nudging a
hostile world into more Earth-like conditions, and we here use the
more general phrase "planetary engineering" (Sagan 1961,1973).
The traditional objective of planetary engineering is to generate an
Earthlike environment on other worlds of the solar system, so that
humans and other inhabitants of the Earth can live there without
special protective gear (spacesuits, large enclosed habitats, etc.).
A continuum of intermediate cases exists, usually more readily
achievable, between the current environment of an extraterrestrial
body and present Earth ambient conditions.
An important special case for planetary
engineering is to reverse significant perturbations (e.g., global
warming) in the environment of our own planet.
This is surely easier
than planetary engineering on other worlds: the circumstances under
which humans can survive unaided represent at most very small
variations from present Earth ambient conditions; but many microbes
and plants grow under less severe constraints (cf. Table I).
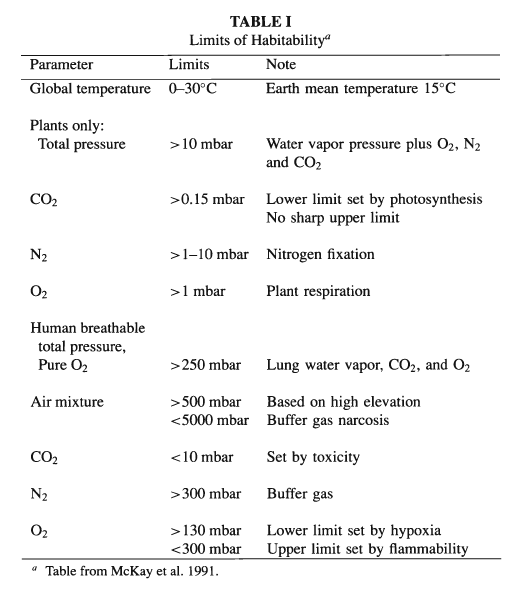
In this chapter we examine the likelihood of accomplishing
significant planetary engineering, particularly towards more
Earth-like conditions, on Mars, Venus and Titan, although
opportunities exist for a number of other worlds in the solar system
as well.
We focus chiefly on proposals by which clement temperatures
(0-30°C) can be realized on a global scale.
Such a temperature range
meets many essential requirements for habitability, including the
potential availability of surface liquid water. As stressed by Sagan
(1961) and by McKay et al. (1991), introducing plants first may lead
with comparatively little additional effort to other desired changes
(e.g., production of significant quantities of photosynthetic O2).
At the end of the chapter we address schemes for countering or
mitigating global warming on Earth.
We adopt the spirit of Henry
David Thoreau's remark,
"What is the use of a house if you haven't
got a tolerable planet to put it on?"
We postulate a future with abundant heavy-lift vehicles,
commercially available and safe nuclear fusion, major practical
advances in genetic engineering and a dedication to the exploration
of the solar system.
Over the next century all of these developments
seem plausible, although they are by no means guaranteed. We also
assume that fusion power will provide us with the technology to move
at will asteroids ten kilometers in diameter and smaller around the
inner solar system. The energy requirements, as we discuss below,
may by then be wholly within reach.
Transport of small worlds within
the inner solar system has long been proposed for other reasons -
e.g., supplying large quantities of precious metals - but much
smaller worlds would suffice for that purpose. This technology may
be developed for other reasons as well: to deflect errant near-Earth
objects from impact trajectory with our planet (cf. Morrison et al.
1992).
However, such technology would also give its possessors an
unprecedented ability to destroy life on Earth, and it is not clear
that a cost/benefit analysis favors its development (Sagan 1992), at
least in the near future.
But in keeping with the optimistic
assumptions of this chapter, we postulate a future in which adequate
safeguards against the misuse of this technology exist.
We assume that fusion power is based on either reactions between
deuterium and tritium (D-T) or between deuterium and 3He (D-He).
This choice is based on the extensive work done over the last
several decades in developing practical schemes for fusion power
(D-T) and plausible projections (D-He) (Cordey et al. 1992;
Wittenberg et al. 1986).
Because of the very low reaction cross
sections of the p-p cycle that helps power the Sun, we ignore the
much more efficient fusion power based on this cycle. In the case of
D-T fusion, 6Li may provide the source of the tritium through
reactions occurring in the blanket of the fusion chamber (Cordey et
al. 1992). For D-He fusion, 3He may be derived from solar wind
implanted in the regolith of the Moon (Wittenberg et al. 1986), or
asteroids.
We assume an adequate amount of 6Li and 3He for the
scenarios considered in this chapter. We do, however, provide
estimates of the amount of water required, which serves as the
source of D.
For D-T fusion, about 20 times the mass of rock is
required to provide the needed 6Li as the mass of water required to
obtain a given amount of D. In either case, the amount of energy
released per mole of reactant is about the same. Below, we assume
that 50% of the mass deficit between reactants and products is
available as useful energy.
Thus, we use a fusion power of 4.2 x
1018 erg g-1 of D or 7.5 x 1013 erg g-1 of H2O, assuming a
terrestrial D/H ratio of 1.6 x 10-4. Utilizing Martian water may
increase the yield per gram of H2O by as much as a factor of 5
because of the higher D/H ratio of water vapor in the Martian
atmosphere.
We do not consider the possible use of thermonuclear weapons for
planetary engineering, because the Outer Space Treaty of 1967 -
which the United States, the Soviet Union (and presumably its
successor states), the nations of Western Europe and Japan, among
many other nations, have ratified - specifically forbids
installation of such weapons on other celestial bodies, and in
"outer space" in general.
The 1963 Limited Test Ban Treaty also
prohibits the explosion of nuclear weapons anywhere except beneath
the surface of the Earth (A.C.D.A. 1982).
It seems possible (Sagan 1986) that between increasing the abundance
of greenhouse gases and placing fine particles at high altitudes to
provide an anti-greenhouse effect, we will have the means to heat or
cool a planetary surface at will; and that, for the latter, it might
suffice to pulverize a small asteroid or cometary nucleus. In
addition, large partially opaque sunscreens emplaced between the Sun
and a given world could be used to cool that world, and fusion
technology could be used to warm it.
In principle, anticipated
advances in human technology might, within the next century, provide
us with practical planetary thermostats.
We take particular note of the time scale for planetary engineering
implicit in various methods. It would seem foolish to implement
today a scheme that would occupy 104 or 106 yr, say, to achieve its
effects, when in, say, 100 yr we will likely have much more powerful
technologies for the purpose. Current political realities suggest
that an expensive project without a significant payoff in 25 yr or
less is infeasible.
As a rough figure of merit, any planetary
engineering scheme that takes more than several decades to achieve
significant results (if not full implementation) should be rejected
on such grounds alone.
Projecting technological trends more than a few decades into the
future is in any case a forlorn exercise. But advances in genetic
engineering far beyond what we assume in the remainder of this
chapter seem possible. We therefore note that, at some time in the
future, a much more elegant way to overcome our parochial habitat
restrictions may be to genetically engineer humans for other worlds
than to physically engineer other worlds for humans (cf. Stapledon
1948).
We find below that planetary engineering generally entails severe
ancillary environmental costs.
This may be because we have not yet
conceived of the really elegant methods.
But these costs,
especially, raise a number of issues:
-
Given that any planetary
engineering scheme entails a balance of benefits against costs, how
certain must we be that key scientific information will not thereby
be destroyed?
-
How much understanding of the world in question do we
need to have before planetary engineering can be relied upon to
produce the desired end state?
-
Can we guarantee a long-term human
commitment to maintain and replenish an engineered world when human
political institutions are so short-lived?
-
If a world is even
conceivably inhabited - perhaps only by microorganisms - do humans
have a right to alter it?
-
What is our responsibility to preserve the
worlds of the solar system in their present wilderness states for
future generations - who may contemplate uses that we are today too
ignorant to foresee?
-
These questions may perhaps be encapsulated
into a final question: can we who have made such a mess of this
world be trusted with others?
At the end of this chapter, we briefly
re-address some of these issues.
II. MARS
Today
Mars is a frigid, desert planet.
The fact that conditions seem
to have been warmer and wetter 4 Gyr ago when the solar luminosity
was ~0.75 its current value (Sagan and Mullen 1972; Pollack et al.
1987) suggests that it may be possible to move the Martian climate
into a more temperate range.
Although today temperatures near the
equator can rise above the freezing point of water during the
warmest times of the day, the diurnally averaged temperatures are
everywhere well below freezing. Here, we consider ways of increasing
the globally, diurnally, and seasonally averaged temperatures to
values comparable to the annually averaged temperatures at
mid-latitudes of Earth.
We note from the start that even if this
were done gently, it would disturb or destroy some major Martian
landforms - e.g., the polar laminae.
The low mean temperatures on Mars are due both to it being further
from the Sun than the Earth (a unit area on Mars absorbs roughly
half the solar flux as an identical area on Earth) and to Mars
having a very thin atmosphere - so that the greenhouse warming is
only about 7 K, in contrast to about 33 K for the
Earth.
On present-day Mars, CO2, the major constituent of the
atmosphere, is the chief agent responsible for its modest greenhouse
warming, through the opacity it provides to thermal radiation
emitted by the surface in the wavelength region around 15
µm.*
*
µm
=
Micrometre
The apparently most cost-effective way
to warm Mars is by enhancing the atmospheric greenhouse effect
through the introduction of gases that absorb at thermal wavelengths
outside the 15 µm region.
There are
two possible sources of such gases - exogenous to Mars and
endogenous. We could imagine, for example, transporting ice from the
outer moons or rings of Saturn to Mars, but pure water, which
appears to be the composition of the rings of Saturn, while an
important greenhouse gas on Earth, would be ineffective on Mars,
because the temperatures there are so low that water freezes out.
In
general for both Earth and Mars, the water abundance is buffered by
reservoirs at the surface, so it can only amplify the greenhouse
effect of other gases once they produce significant warming. We
require gases that not only are effective infrared absorbers, but
that also remain in the gas phase at present Martian temperatures.
Below we discuss three candidates, NH3, CO2 and halogen compounds.
-
Ammonia
It would be far more effective to utilize materials already on Mars.
NH3 absorbs strongly both near 10
µm and longwards of 20
µm.
Small
amounts can greatly enhance the greenhouse effect on Mars.
Calculations suggest (Sagan and Mullen 1972; Pollack 1979) that a
partial pressure of
ammonia of ~10-4 bar is needed to warm the
Martian surface to above the freezing point of water.
The required amount of NH3 might be generated in several ways.
First, consider appropriately designed microorganisms that convert
atmospheric N2 to NH3, an exothermic reaction, with the hydrogen
derived from subsurface bound or frozen water. Nitrogen-fixing
microorganisms are abundant on Earth and central to agriculture;
they routinely convert N2 to NH3.
But none is known to metabolize
under simulated Martian conditions.
Cryophilic nitrogen-fixers that
extract their water requirements from bound or solid water would
have to be engineered; and/or heated artificial habitats created
that would vent NH3 to the outside atmosphere. As the partial
pressure of N2 on Mars is ~2 x 10-4 bar, about 25% of the available
atmospheric nitrogen would be required to generate 10-4 bar of NH3.
Properly engineered microorganisms might be placed on the permanent
water ice north polar cap. Despite the summertime temperatures of
about - 30°C, the solar flux might be sufficient for such
microbes to provide photosynthetically the energy needed to
manufacture ammonia.
Alternatively, ammonia might be generated by chemical factories that
use nuclear fusion as a power source to convert atmospheric N2 to
NH3. The hydrogen needed both for fusion fuel and for the chemical
conversion of N2 to NH3 could come from the residual north polar
cap, or perhaps from the regolith.
If as much as 500 calories of
activation energy were needed to convert each N2 molecule to NH3,
~1.7 x 1013g of water for a terrestrial D/H ratio would be needed for
fuel to produce 10-4 bar of NH3 over the entire planet. (Even less
water is needed if the observed Martian atmospheric D/H ratio is
used).
This corresponds to only about
the top 40 µm of the north
polar cap. However, about 30 cm of H2O from the north polar cap
would be consumed in supplying the H needed to convert 1/4 of the
current atmospheric N2 to 10~4 bar of NH3.
As a third possibility, especially if the budget of atmospheric N2
and surface nitrates is not quite adequate to generate T >0°C
through an ammonia greenhouse, N2 might be derived from the
atmospheres of Venus, Earth, or Titan and transported to Mars.
For
example, to generate an NH3 partial pressure of ~1 x 10-3 bar,
amounts of N2 equal to ~5 x 10-4, 1 x 10-3, and 5 x 10-5 times the
N2 content of the atmospheres of Venus, Earth, or Titan,
respectively, would be required. If liquefied, the requisite amount
of N2, ~6.8 x 1017 g, would measure about 10 km across.
If a ∆V of
10 km s-1 were needed to transport the liquefied N2 to Mars, ~4.5 x
1015 g of H2O would be required to power the fusion engine. This is
equivalent to the amount of water in a 1-km-sized comet.
Alternatively, the N2 might be derived from the N in organic
compounds contained in C or D asteroids, whose orbits come close to
that of Mars.
Such bodies may contain ~0.5 wt% N. Thus, a much lower
∆V might suffice for transporting nitrogen to Mars. An asteroid of
diameter about 50 km would be needed to supply the requisite amount
of N. The cheapest, although least responsible, method of extracting
the nitrogen would be by simply crashing the asteroid into Mars;
this would generate a crater hundreds of km across, destroying the
underlying terrain.
There is, though, an important problem with any scheme to use NH3 as
a Martian greenhouse gas: ammonia is readily dissociated by solar
ultraviolet radiation at wavelengths, X, shortward of ~0.23
µm
(see, e.g., Atreya et al. 1978).
In the Martian atmosphere, neither
CO2 nor H2O would block ultraviolet radiation at k>0.2
µm. A net
dissociation rate of only ~50%, a reasonable lower bound, implies
~2.4 x 1012 molecules dissociated cm-2 s-1 at Mars (ibid.). Thus,
10-4 bar of NH3 would be totally converted back to N2 by solar
ultraviolet radiation in about 30 yr.
However, 30 yr is much longer than the radiative time constant for
the Martian atmosphere; so before NH3 is appreciably
photodissociated, its infrared opacity will have significantly
warmed the surface. Thereafter, the gas phase dissociation products
of NH3, N2 and H2 would be recycled to NH3 by essentially the same
technology used to produce it initially.
Another potential problem
is the reaction of NH3 with atmospheric CO2 to form ammonium
carbonates and other salts at the low temperatures of the winter
polar regions; but the kinetic time scales are unknown for these
compounds, and any that form in winter may be vaporized the
following spring.
-
Carbon Dioxide
A second possible way to augment the greenhouse effect on Mars is to
increase the
carbon dioxide content of its atmosphere.
At
sufficiently high pressures, pressure-induced transitions and hot
bands of gas phase CO2 close the thermal infrared windows. Clement
average temperatures can be achieved by raising the surface pressure
from its current value of 7 mbar (millibars) to ~ 1 bar (Pollack et
al. 1987).
One conceivable source of atmospheric CO2 might be the dry ice
contained in the polar caps. However, the large perennial cap in the
northern hemisphere is composed, apparently exclusively, of water
ice.
The smaller perennial cap in the south had a surface layer of
CO2 ice during the first year of the Viking spacecraft mission
(1976-77), but in other years this layer may be very thin or even
disappear. An upper limit to the amount of CO2 available in the
Martian polar caps can be derived from the fact that under high
enough hydrostatic pressures, solid CO2 will liquefy; this limit
corresponds to a cap thickness of ~2 km (Sagan 1973).
Even if all
the south cap were CO2 to a depth of 2 km and even if all of this
carbon dioxide were liberated in the gas phase, it would amount only
to some 60 mbar pressure when uniformly distributed over the planet.
Thus, the available CO2 from the polar cap falls short of the
required abundance by more than an order of magnitude.
If, nevertheless, it were desirable to vaporize the dry ice in the
polar cap to begin increasing CO2 partial pressures, we might
consider lowering the cap's
albedo by depositing very dark
carbonaceous material of asteroidal origin, or genetically
engineering plants with dark, broad leaves that can survive on the
polar caps (Sagan 1973).
Another source of CO2 is carbonate anions in the rocks.
Spectroscopic observations of Mars provide evidence for significant
amounts of carbonates (Pollack et al. 1990).
Suppose that these
rocks in toto contain more than a bar of CO2 and that they are
preferentially concentrated in certain basin areas. If nuclear
fusion were used as a power source to liberate this CO2, then ~5.7 x
1017 g of H2O would be required to generate 1 bar of CO2 -
equivalent to about the top meter of water in the north polar cap.
This solution for planetary engineering of the Martian climate,
while much more energy-intensive than the NH3 solution, has the
advantage of being much more permanent. CO2 would not be converted
to some other gas species by solar ultraviolet light and would only
very slowly be transformed into carbonate rocks by water-abetted
weathering of surface rocks.
The latter process would have a time
scale of about 10
Myr (Pollack et al. 1987).
If the spectroscopically derived carbonate abundance is
approximately correct and representative of the top several km of
crust, the entire surface of Mars would have to be plowed up and
processed to a depth of 2 km in order to generate a 1-bar CO2
atmosphere.
Apart from the daunting obstacles in practical
engineering that this represents, it would also constitute the
irresponsible destruction of a unique scientific resource and
database - the Martian surface. If carbonate deposits are
concentrated preferentially (by, say, an order of magnitude) in
basins, then the basins would have to be excavated to a considerable
depth - better, at least, than bulldozing the entire planet.
We find that both environmental ethics and probable cost argue
against large augmented greenhouse effects from indigenous CO2.
-
Chlorofluorocarbons (CFCs)
A number of
CFCs have vibrational fundamentals that lie within the 8
to 13µm window region of the atmosphere, and have been suggested as
a significant potential agent for global warming of Mars (Lovelock
and Allaby 1985; McKay et al. 1991).
At current Martian
temperatures, though, these absorption bands would cause only a
small amount of greenhouse warming, because they lie on the
short-wavelength tail of the Planck blackbody function. But they
would give rise to a more substantial greenhouse effect as the
surface temperature approaches 273 K due to other greenhouse gases,
and the Wien peak of the blackbody function shifts to shorter
wavelengths.
In principle, CFCs could be much more potent in significantly
elevating the present Martian surface temperatures if they had pure
rotational spectra that began near 20
µm and extended to longer
wavelengths, as does NH3. To do so, they must have permanent dipole
moments and large moments of inertia. We suspect that HCFCs (e.g.,
CHCI2F) might meet both these requirements. Unfortunately, we are
not aware of any relevant laboratory measurements at the long
wavelengths of interest. We defer a discussion of the source of CFCs
until the next subsection.
For all greenhouse gases, the temperature increase would occur
essentially on the time scale of release of the corresponding gases:
the thermal time constant of the present Martian atmosphere is
around 2 days; for ~ 1 bar CO2, it would be around 300 days, still
very short compared to the other time scales of the project.
As
temperatures approach the freezing point, water vapor partial
pressures in the atmosphere would increase, water vapor would
augment the greenhouse effect due to the other gases, and the final
stages of reaching clement temperatures might become comparatively
easy.
Direct fusion heating of the Martian surface from power plants
distributed over its surface is briefly described in the section on
Titan below.
-
Possible Completion Scenarios
So far we have focused exclusively on schemes for increasing the
mean global surface temperature of Mars to above the freezing point
of water, i.e., into a habitable temperature regime.
We now consider
scenarios by which Mars is made habitable in a more complete sense
for microbes, plants, other animals, and humans, with Table I as our
guide for what needs to be done to the atmospheric composition to
achieve this ultimate goal. Here we draw heavily upon the discussion
of McKay et al. (1991).
The first human settlements on Mars will result from much more
modest planetary engineering. We imagine these settlements to be
small, enclosed microenvironments, in which the conditions of Table
I are met. From them, in time, flows the means for generating more
and larger microenvironments, culminating in a full-scale remaking
of the entire planet's climate into one suitable first for microbes
and plants, and later for widespread habitation by animals,
including humans.
How might this be brought about?
McKay et al. (1991) have suggested that the first milestone, that of
habitation by macroscopic plants, could be achieved by priming the
greenhouse pump and allowing for natural amplification mechanisms to
greatly increase the resulting warming. In particular, they
recommend introducing enough greenhouse gases to warm the surface by
about 20 K above present ambient.
Such warming, they suggest, will
lead to a large out-gassing of CO2 from the regolith, where it is
adsorbed onto grain surfaces, and from the small perennial south
polar cap. In this way the CO2 pressure may be increased to a value
as high as 100 mbar. Additional release of CO2 might either occur
from regolith or polar CO2 reservoirs, or be accomplished by
releasing CO2 from carbonate rocks, until a CO2 pressure of a bar to
a few bars was achieved, at which point surface temperatures would
permit survival and growth.
However, we believe it is unrealistically optimistic to think that
any significant fraction of one to a few bars of CO2 could be
released from the regolith or polar regions, based on what is known
about the nature of the Martian surface (see above).
Furthermore,
even 100 mbar of CO2 would not significantly enhance the surface
temperature (Pollack et al. 1987). Therefore, this scenario must
draw heavily upon the release of CO2 from carbonate rocks. In this
regard, fusion power may play an essential role because of the very
large amount of energy released per gram of water, as illustrated in
our earlier discussion in this section.
However, as discussed above,
it will still be a highly nontrivial task to generate the requisite
amount of CO2 from carbonate rocks. The introduction of much more
modest amounts of NH3 or hydrogenated CFCs appears to offer a much
more viable approach to raising the surface temperature of Mars into
the habitable regime.
According to Table I, several chemical
modifications of the Martian atmosphere would be needed to make the
planet habitable for plants, apart from the greenhouse warming
discussed above. Here too, fusion power may prove to be very useful
by helping to generate modest but enhanced partial pressures of O2
(e.g., by conversion of small quantities of CO2) and N2 (e.g., by
conversion of nitrates).
Once this milestone is achieved, the second stage - making Mars
habitable for animals and for humans - can be addressed (cf. Table
I). McKay et al. (1991) suggest that plant photosynthesis could
convert enough atmospheric CO2 to O2 to meet the oxygen
requirements.
However, this suggestion rests on a misunderstanding,
as the O2 released by green plants derives exclusively from H2O and
not from CO2. Such a photosynthetic source of O2 would require at
least 260 mbar of H2 (which need not all be in the atmosphere at the
same time) and 130 mbar of CO2.
Alternatively, fusion power could
extract the needed oxygen from the highly oxidized surface, although
several meters of regolith would need to be converted on global
average. In addition, an enormous amount of N2 would need to be
introduced into the atmosphere to buffer the O2 and prevent ready
combustion from occurring.
It is not clear that Mars has the
requisite amount of N in easily accessible reservoirs.
The
importation of the needed amount of N2 from elsewhere in the solar
system would be a formidable task, as illustrated by our discussion
of obtaining a much more modest amount of N for generating NH3.
Finally, the relative abundances of greenhouse gases would need to
be carefully adjusted. Partial pressures of CO2 greater than MO mbar
are toxic for humans. Thus, the CO2 pressure might need to be
greatly reduced from its earlier value, and CO2 could not a
prominent role in the late stages of greenhouse warming for human
habitability.
Likewise (NIOSH/OSHA 1978; Sittig 1985), 0.05 mbar of
NH3 is the short-term inhalation limit for humans at 1 bar (although
some microbes can survive much higher NH3 mixing ratios), which is
comparable to the NH3 partial pressure needed for it to become the
dominant greenhouse gas.
Note that the required NH3 partial pressure
decreases from ~0.1 mbar to a much lower value as the total pressure
increases from 7 mbar to ~1 bar, so it seems possible to engineer a
significant NH3 greenhouse effect without reaching toxic levels.
Alternatively, CFCs or related compounds which have very low
toxicity and ultraviolet liability, might serve as the chief
greenhouse gases in completion scenarios, but subject to the
far-infrared absorption considerations cited above. Manufacture on
Earth of enough CFCs to warm Mars can be readily foreseen, because
in only a few decades with present technology we have managed to
synthesize enough to contribute to global warming on our planet.
Transportation to Mars would be
expensive: using Saturn V or Energia
class boosters would require at least a launch a day for a century.
The manufacture of sufficient quantities of CFCs and allied
substances on Mars from evaporites or igneous fluorite or fluorospar
might be feasible (J. Lewis, personal communication, 1992; Fogg
1992).
In either case, as the CFCs would be photolytically destroyed
in about a century, judging from the situation on Earth, this
delivery or manufacturing rate would have to be continued forever,
although recycling procedures would become the method of choice.
However, CFCs as a principal greenhouse gas on Mars have a different
undesirable property: they inhibit the formation of a substantial
ozonosphere. The temperatures may be made clement, but the solar
ultraviolet flux would still pose a very serious hazard. Perhaps
this in turn could be fixed with a high-altitude layer of
ultraviolet-absorbing particles, but the difficulty of planetary
engineering on Mars is thereby greatly compounded.
Perhaps some
nontoxic, long-infrared-absorbing, ozone-non-interactive, and
preferably inexpensive gas phase molecule that would do much better
than CO2, NH3 or the CFCs remains to be synthesized. A more
promising and less hypothetical alternative is to employ bromine-
and chlorine-free halocarbons or related compounds (e.g., SF6); F is
much less effective in destroying O3 than are CI and Br (see, e.g., Fogg 1992; Chamberlain and Hunten 1987).
Thus, NH3 along with F
compounds appear to offer the most promising means of warming Mars
for habitation by humans.
In summary, it appears possible, although difficult to bring Mars
globally to clement mean surface temperatures, more difficult to
create conditions suitable for plant habitation, and much more
difficult to create conditions necessary for unprotected humans to
live there.
Even with rather optimistic assumptions on the progress
of technology over the next century, and with very optimistic
assumptions about the cost we will be willing to pay, it is not
entirely clear that extensive planetary engineering of Mars is
within reach.
III. VENUS
Because of its proximity to the Sun and its massive atmosphere, a
very effective greenhouse operates on Venus that raises its surface
temperature from what it would be without an atmosphere (about 240 K
with the present albedo) to about 730 K.
CO2 is the major
constituent of the Venus atmosphere and its opacity is the chief
source of the greenhouse effect (Sagan 1960; Pollack et al. 1980).
As the CO2 pressure at the surface is ~90 bar, equivalent to ~105 g
cm-2, engineering Venus by eliminating almost all its atmospheric
CO2 would appear to be a formidable undertaking.*
* If we could wholly
remove other greenhouse gases such as H2O and
SO2, we could lower the surface temperature by hundreds of °C, but
still not nearly enough to fall below the normal boiling point of
water (cf. Pollack et al. 1980). However, if the CO2 abundance were
massively reduced, pressure-broadening of H2O and SO2 would be
greatly diminished, and their infrared opacity would become
comparatively small.
We say "almost,"
because some residual greenhouse effect might be needed to bring the
temperature from about 240 K to about 300 K.
Alternatively, such
fine tuning might be provided by adjusting the surface
albedo or the
albedo of high-altitude aerosols.
-
Impact Erosion
As a calibration of this formidability, we consider bombarding the
planet with large, asteroid-sized bodies (tens to hundreds of km in
size) - recognizing that such bodies may have been effective in
eroding the atmospheres of the terrestrial planets, especially
during the first billion years or so of their history when impact
fluxes were much higher (Walker 1986; Melosh and Vickery 1989).
High-velocity collision with the solid surface of a planet releases
the kinetic energy of the impactor on a very short time scale.
Several tens of percent of this energy is released into the
atmosphere near the impact site as hot rock vapor, generating a
shock wave that propagates outwards through the atmosphere.
When the
energy in the shock and vapor plume exceeds the gravitational
binding energy of the portion of the atmosphere it traverses, this
portion of the atmosphere can escape to space. For all but perhaps
the largest impactors, the spherical geometry of the planet limits
the affected portion of the atmosphere to the local tangent plane,
corresponding roughly to 3 x 10-4 of the total volume of the
atmosphere.
According to calculations by Vickery and Melosh (1990),
silicate bodies having masses -5 x 1018 kg (corresponding to objects
larger than 150 km in diameter), and velocities in excess of ~20 km
s-1 are able to blow away all of the Earth's atmosphere above the
tangent plane.
The atmospheric mass dissipated falls rapidly as the
a mass of the impacting object diminishes, with no loss occurring for
objects smaller than ~2 km in diameter, regardless of impact
velocity. Also, no loss occurs when the impacting velocity falls
below 20 km s-1, regardless of the impacting body's mass. Similar
thresholds apply to cometary impactors, although limited blowoff can
occur at velocities somewhat below 20 km s-1.
Because the escape velocity for Venus is similar to that for the
Earth (10.4 vs 11.2 km s-1), very similar limits on the minimum
velocity needed to cause blowoff apply there.
However, the mass
requirements are increased by about a factor of 100 because of the
greater atmospheric pressure at Venus' surface. Thus, impactors
larger than ~10 km are required to produce any blowoff and ones
larger than ~700 km are needed to blow off all the atmosphere above
the tangent plane.
To remove most of Venus' atmosphere through
impact erosion would therefore require about 3000 impactors each
larger than 700 km and traveling at velocities in excess of 20 km
s-1. There is nothing like this number of large bodies in the solar
system, and if there were, it would surely be irresponsible to
destroy them all (to say nothing of the present surface of Venus).
Even with fusion power, excessive amounts of energy would be needed
to accomplish the atmospheric removal.
We conclude that impact
stripping of the Venus atmosphere is an ineffective approach to
planetary engineering.
-
Microbes
A much less energy-intensive and gentler approach is the
introduction of genetically engineered micro-organisms into the
clouds of Venus (Sagan 1961), where moderate temperatures prevail
and where there is some water available.
They would fix CO2 into
involatile organics or graphite, undoing much of the greenhouse
effect, and lowering surface temperatures so that the sparse
atmospheric water vapor would condense out on the surface in pools
and shallow seas. Very special micro-organisms would be required
because we now know the clouds of Venus to be a 75% solution of
concentrated sulfuric acid which, among other disabilities, is a
powerful desiccant.
Nevertheless, there are micro-organisms known
that live in concentrated solutions of H2SO4 and die in pure water
(see also Seckbach and Libby 1969). We assume an H2SO4-tolerant
N2-fixing green plant photosynthetic micro-organism that could
survive and replicate in the clouds of Venus.
Biological solutions
to planetary engineering have the advantage that microorganisms can
rapidly replicate in the right environment; only a comparatively
small number of such microbes then need be introduced.
This approach has been criticized for ignoring the fact that for
every mole of CO2 consumed in photosynthesis, a mole of water vapor
is also required. As there is 3 x 10-5 as much H2O in the Venus
atmosphere as CO2, it has been suggested that the process would
grind to a halt far short of the desired transformation.
However,
the original proposal addressed this issue: photosynthesis goes
according to the standard heuristic equation,
...in which the O2 derives exclusively from the water. The
carbohydrates thus synthesized will be circulated to the deeper
hotter layers of the Venus atmosphere and pyrolyzed:
The carbohydrate is reduced to elemental carbon, or something
approaching it, and the water is released, circulated back to the
upper atmosphere and available for the next round of chemical
reactions.
The net chemistry is then approximately
There are two critical questions:
-
whether all (within 3 x 10-5)
of the carbohydrate hydrogen is released at depth and recirculated
-
whether the kinetic barrier to graphite oxidation will be
maintained at the temperatures of the middle and lower atmosphere of
Venus
These are matters accessible to laboratory experimentation
and thermodynamic equilibrium-kinetic calculations.
Preliminary
evidence on (2) is unfavorable: everyday experience with
self-cleaning ovens suggests that re-oxidation of carbohydrate pyrolysis products to CO2 at 700 K and 0.2 bar O2 occurs on a time
scale ~ 1 nr.
Even if a microbiological approach to engineering Venus were
feasible, however, the surface would be buried by hundreds of meters
of fine graphite or organic particles sedimenting out of the
atmosphere; and ~65 bars of O2 would be generated - the first at
least inconvenient for, and the second lethal for human settlers on
an engineered Venus.
Such microbiological schemes at best carry
planetary engineering on Venus only part-way towards human
habitability.
-
Anti-Greenhouse Effect
Greenhouses can be turned down or off by substantially reducing the
amount of sunlight reaching the surface, as in nuclear winter (Turco
et al. 1983; see also, Hoyle and Wickramasinghe 1978).
In the case
of Venus, this might be accomplished, despite the resulting lowered albedo, by introducing a planet-wide layer of highly absorbing
particles into its upper atmosphere. Naturally, this layer will
slowly sediment towards the lower atmosphere and ground, and so
would need periodic replenishment.
Elemental C is a very good absorber of sunlight. Taking a typical
value of 0.3 for the imaginary part of its refractive index (Turco
et al. 1983), we find that particles of radius 0.1
µm or more
absorb almost all of the visible light passing through them.
Particles of such sizes have an interaction cross section comparable
to their geometric cross section at mid-visible wavelengths.
Small
particles are desirable for this scenario because,
-
the total mass
required for a given amount of solar attenuation varies as the mean
particle size (as long as the interaction cross section is
comparable to the geometric value
-
their fall velocity
varies inversely (as the first or second power) as their size
If a particle radius of 0.1
µm is assumed and an elemental C particle
absorption optical depth of 5 is needed to diminish adequately the
amount of sunlight reaching the Venus surface, then ~6 x 1014 g of
carbon are required.
This is equivalent to a single 1-km-sized
asteroid made of carbonaceous chondritic material. Particles 0.1
µm
in radius would take several hundred years to fall to the surface at
their sedimentation velocity (see, e.g., Ryan 1964), but might
actually have lifetimes of only a few years when allowance is made
for vertical transport by winds at a variety of length scales.
Carbon-rich (especially C- and D-type) asteroids that cross the
Venus orbit might serve as the source of the carbon needed to create
an anti-greenhouse effect on Venus. Orbital velocity changes needed
to transport the asteroidal material to Venus are ~ 10 km s-1,
corresponding to ~5 x 1011 erg g-1 elemental C transported.
Note
that this specific energy is much larger than that needed to produce
C from organic compounds found in asteroids. If once again fusion
power is used, 1 g of H2O would be required to transport about 2 x
103 g of C or a total of 2 x 1012 g of H2O would be needed to create
the opaque particle screen. We neglect the energy cost of
pulverization and distribution of fine particles.
This is clearly a
low-mass, low-energy option for transforming Venus.
There are, however, several disadvantages to the anti-greenhouse
option. It is still expensive in asteroids - requiring the periodic
pulverization of a 1-km-sized organic-rich, water-rich asteroid.
The
thermal response time of the Venus atmosphere is a few decades, so
if the high altitude layer of sedimenting opaque particles is not
replenished, the carbon dioxide greenhouse effect reasserts itself
on such a time scale and the surface temperature rises again to ~730
K.
In addition, by its very nature, this option plunges the surface
of Venus into deep gloom, with ambient light levels in daytime
perhaps only as bright as on a moonlit night on Earth, and the
oppressive 90 bar atmosphere remains untouched. This might
conceivably be acceptable for an exploratory mission with human
crews, but seems rather stark for a self-sustaining community of
people on Venus.
Further, as for all schemes, settlers would have to
adjust to the very unearthlike 243 day sidereal period and 125-day
synodic period (sunrise to sunrise).
-
Sunshades
Another approach is to construct a system of sunshades, perhaps of
adjustable opacity, in space around Venus (Dyson 1989; Birch 1991).
Alternatively, we might place the sunshade at the first Lagrangian
point, Li. The most efficient such sunshield, with sunlight assumed
incident in parallel rays, would have a shadow the size of the
object casting it.
Were the shield as thin as 1
µm, its mass would
be ~1014 g. But for Venus to be in the umbra rather than the
penumbra of the shadow cast, the shield would have to be roughly an
order of magnitude larger and, therefore, have a mass ~10 g. We thus
run into apparently daunting economic problems, as discussed below
for the Earth.
With sunshades the Venus surface temperature could be
brought to very low values; Dyson (1989) and Birch (1991) even
discuss the possibility of freezing out the atmospheric carbon
dioxide into CO2 oceans or glaciers.
-
Chemical Transformations
Birch (1991) further suggests that the condensed CO2, when in the
liquid phase, would flow to the lowlands, forming CO2 oceans.
He
then advocates covering the CO2 seas to prevent their
re-evaporation, bringing a several hundred km ice-rich moon from the
outer solar system to form water oceans on top of the covered CO2
seas, and only partially blocking the sunlight to establish an
Earth-like temperature at the surface.
Finally, a
soletta (a
sunshade that opens and closes) could create a 24 hr day/night
cycle, while the establishment of plants on land could generate O2
from a residual amount of CO2 in the atmosphere (perhaps leaked from
the CO2 oceans).
From a conceptual point of view, the Dyson/Birch proposal comes
closest to making Venus into a habitat for plants, animals and
people. One could imagine adjusting the amount of solar blockage
during the early phases of the project so that CO2, but not N2
condenses out of the atmosphere, with a view of meeting the N2
requirements of Table I.
However, this proposal involves a massive
engineering effort (e.g., to build the sunshade and to cover the CO2
seas), far beyond anticipated human resources during the next
century. Furthermore, preventing the leakage of CO2 back into the
atmosphere once an equable climate is established would be a
formidable task: at room temperature, essentially all the CO2 sea
would become vapor. This sea would cover most of the Venus surface
area.
Could leaks be prevented from occurring?
A lower-energy method of removing the atmospheric CO2 would be to
convert it to carbonate rocks, because the net reaction is
exothermic. However, this approach (whether microbiological or
industrial) is complicated by considerations of the*mass of reacting
(and pulverized) materials needed.
The converted CO2 would form a
layer 400 m deep over the entire surface area of the planet, and an
equivalent depth of CaO, MgO, or other suitable materials for
forming carbonates would have to be processed. Again, many surface
features would have to be not just covered, but obliterated.
Nevertheless, let us examine the prospects of converting almost all
the CO2 to low vapor pressure materials. Two ways to do this have
been suggested. Birch (1991) proposes importing massive amounts of
Ca and Mg as metals from Mercury. These metals would first reduce
CO2 to C and their metal oxides.
The oxides would then react with
CO2 to produce carbonates. To gather enough metals from Mercury,
however, would require processing M).5% of the planet's mass. Birch
imagines that this would be done by self-replicating robots.
Alternatively, J. Lewis (personal communication, 1992) has suggested
that hot liquid water circulating through the near-subsurface of
Venus would chemically weather the crust (due to dissolved CO2 in
the ground water, forming carbonic acid), leading to the formation
of alkali metal and alkaline earth carbonates (see also Gillett
1991).
A similar process occurs at room temperature on Earth and is
a major component of the long-term geo-chemical C cycle (cf. Pollack
et al. 1987).
One could imagine the following scenario by which
Lewis' scheme is used to create habitats on Venus:
Next, the
amount of sunlight reaching Venus' surface is somewhat reduced -
either by using a sunshade or by introducing a limited amount of
dark absorbing particles into its high atmosphere.
Hot liquid water
now is present at the surface and greatly accelerates the weathering
rate of atmospheric CO2, leading eventually to the elimination of
almost all the atmospheric CO2.
At this point, clement temperatures
can be established and maintained by keeping the amount of sunlight
reaching the surface somewhat below levels that would obtain if
there were no attenuating screens. (Otherwise, a runaway greenhouse
would occur, with the formation of a steam atmosphere [Pollack
1991].)
Now, plants that grow at the comparatively low light levels
can be introduced and the final steps taken to make Venus habitable
for humans.
-
Summary
In summary, making Venus habitable for either plants or people is an
extremely formidable task.
There does not seem to be any elegant way
to do it. The key is to eliminate almost all the CO2 from the
atmosphere. There simply are not enough big bodies in the solar
system to strip away its atmosphere (and even if there were, we
might not want to use them). Atmospheric CO2 might be condensed at
the surface or chemically transformed.
The latter possibility
appears to be more promising in that the CO2 is permanently
sequestered although hundreds of meters of carbonates would now
cover the surface. In addition, there is a need to augment
significantly the water content of Venus' atmosphere and surface
through the importation of water from elsewhere in the solar system.
Finally, a limited blockage of sunlight must be maintained to
prevent a runaway greenhouse from occurring. None of these proposals
seems feasible for the next century.
IV. TITAN
Titan, the largest moon of Saturn, is the only satellite in the
solar system with a substantial atmosphere. Its surface pressure is
~1.5 bar (the column mass density is ten times that of Earth).
The
atmosphere is made mostly of N2, with significant amounts of CH4 (5
to 10% at the surface) and H2 (a few tenths of a percent), as well
as trace amounts of gas-phase hydrocarbons and nitriles. An
optically thick organic smog layer (created by solar ultraviolet
light and Saturn magnetospheric electrons) is present in its
stratosphere.
The surface may contain extensive reservoirs of
organics, and water and ammonia ice deposits.
Currently, the surface
temperature is only about 95 K. A modest greenhouse warming of 10 K
is produced by pressure-induced transitions of N2, CH4 and H2 (McKay
et al. 1989). A major infrared window region, that extends from ~ 17
to 35 µm wavelength prevents a more substantial warming from
occurring.
In principle, Titan's greenhouse could be significantly augmented by
introducing gases that absorb strongly in the window region.
However, the low surface and tropospheric temperatures greatly limit
the vapor pressures of essentially all cosmically abundant potential
candidates (e.g., NH3, CO2 and H2O), which makes this approach
difficult to implement.
Many less abundant organics do not absorb
preferentially in this window region, because their vibrational
fundamentals tend to lie at shorter wavelengths and their rotational
transitions at longer wavelengths. However, there are molecules that
absorb in this region, including some alkanes, alkenes, and amides,
and it might be possible to manufacture substantial quantities of
such molecules from indigenous or exogenous resources.
An alternative approach is to heat the surface directly.
McKay et
al. (1989) have estimated the greenhouse warming that may have
occurred when the sunlight reaching the lower atmosphere and surface
was augmented by accretional heating due to the planetesimals that
helped to build this satellite. There exists a very strong feedback
between total surface flux (sunlight and accretional heating) and
surface temperature, because of the exponential dependence of the
vapor pressures of ammonia and water on surface temperature and the
potential of these molecules for closing the greenhouse windows.
Surface temperatures comparable to terrestrial values can be
achieved when the accretional heat flux reaches ~105 erg cm-2 s-1,
which is ~0.05 the solar constant at Earth.
Clement temperatures on Titan could also be achieved through heat
released by nuclear fusion, with surface volatiles supplying the D
fuel. (We assume that there are substantial deposits of ammonia and
water ice in contact with the atmosphere so these greenhouse gases
may be mobilized. There is no shortage of sources of H.) To generate
a mean heat flux of ~105 erg cm-2 s-1 across Titan's surface (with
widely dispersed power plants), or equivalently 8 x 1022 erg s-1, 2
x 104 g of deuterium would need to be consumed per second.
An equivalent depth across Titan of some
400 µm of H2O (or NH3 or CH4
or C2H6) would be needed for fuel each year (assuming a terrestrial
D/H ratio).
Such a requirement might easily be met and could be
sustained for an extended period of time without exhausting the
supply of H (which is easy) or without doing extensive environmental
damage (which is more difficult).
We also recognize that just the
act of warming Titan to room temperature might cause extensive
modification of its surface, as well as its atmosphere. Indeed, the
nature of Titan's surface after such a warming would be an important
element in determining the desirability of altering its climate.
If
Titan were not habitable or could not be made habitable after this
change, there clearly would be little motivation to do it.
In certain ways, Titan might be the easiest extraterrestrial object
to make habitable. Unlike Mars, there is enough N2 in its atmosphere
to meet the requirements of Table I.
Very likely abundant water
resides at or close to Titan's surface. There is also enough oxygen
in the form of H2O, CO and CO2 to serve as source material for
generating O2. However, the greatly reduced solar flux at Titan's
distance from the Sun (down a factor of 100 from the Earth) would
limit the rate of photosynthesis (and perhaps prevent it from
occurring for some plants).
Also, there is the question of how much
dry land there would be once its surface temperature was raised
above the melting point of water ice. Finally, the abundance of some
atmospheric gases might need to be limited to prevent toxic levels
from being reached (e.g., NH3).
A similar scheme might be considered for Mars, but Titan has several
advantages in this respect: the atmospheric pressure is much
greater, and NH3 and other greenhouse molecules (besides H2O) are
already present in the condensed phase. There is thus a much
stronger greenhouse positive feedback to surface warming on Titan
than on Mars.
We estimate about an order of magnitude more
technological power dissipation is required to bring Mars to 0°C
than to bring Titan, despite the much lower present temperatures on
Titan.
A similar scheme might apply to Neptune's moon Triton if it
has near-surface NH3 ice.
V. EARTH
Our own planet has a uniquely suitable climate for our kind of life
- no coincidence because the environment and the biology have
co-evolved.
However, as mentioned in the introduction, human
technology is swiftly and dangerously altering that environment. We
here briefly consider ameliorating one such potential danger, i.e.,
countering global warming with some form of planetary engineering.
We note immediately that the scale of global warming over the next
century is predicted to be several degrees C, so any solution to the
increasing greenhouse effect must have high precision.
Beyond that,
even if the global mean temperature increase can be slowed or
stopped, we must be careful not to cause local and regional
agricultural and economic disasters in the process. The cure must
not be worse than the disease.
The debate about incompletely
understood and possibly highly nonlinear feedback effects in the
global climate, aired in the greenhouse context (see, e.g., DOE
Multi-Laboratory Climate Change Committee 1990), is a particular
reason for caution.
Such considerations already suggest that
planetary engineering of the sorts discussed above may be
inappropriate for an already inhabited planet.
-
Anti-Greenhouse Effect
In countering greenhouse warming, we might try to reduce slightly
(by a few percent) the amount of sunlight reaching the lower
atmosphere by creating a carefully titrated optically thin particle
layer at high altitudes.
A 1% change in the albedo of the Earth then
buys us about a 1°C change in temperature directly, and about a 2°C
change when we take account of the water vapor greenhouse feedback.
The mass of fine particles required is some 1012 to 1013 g; they
would not seriously diminish the light levels at the Earth's
surface. If they were emplaced in orbit, the required mass would be
the equivalent of some tens of thousands of shuttle launches.
Even
with a substantial improvement in the human species' heavy lift
capability, this seems out of reach for some time. Moreover, the
pollution generated by the required launcher traffic might cause
significant damage to the ozone layer. Alternatively, the fine
particles could be placed at stratospheric altitudes.
As in the case
of Venus, the layer would then have to be replenished about once a
year.
One of the first suggestions on how to mitigate greenhouse warming
was to carry sufficient elemental sulfur to the lower stratosphere,
and to burn it to sulfur dioxide which is then converted into
sulfuric acid droplets which scatter sunlight back to space (Budyko
1974,1977, and references therein; see also Broecker 1985; for a
more general suggestion on cloud condensation nuclei see Latham
[1990]).
Alternatively, SO2 could be carried up directly. A modern
version of Budyko's calculation might go as follows: 1 to 10 Tg (1
Tg = 1012 g) of H2SO4 in small droplets would probably suffice to
nullify a few degree greenhouse warming. This would require the
transport of some 1 to 10 Tg of S or SO2 to the lower stratosphere
every year (assuming one year for the aerosols to fall out), or
equivalently 106 to 107 metric tons per year.
The military transport
aircraft with the largest payloads in the world are
the Russian AN
225, which can lift 250 metric tons, and
the U.S. C-5B which can
lift 125 metric tons (Cheney 1989). Allowing for the apparatus to
oxidize the elemental sulfur at altitude (if SO2 itself is not
carried), and the desire to get to higher altitudes than just above
the tropopause, let us assume aircraft with 100 ton payloads.
This
then requires between 30 and 300 flights per day into the indefinite
future. Budyko points out that the resulting sulfur precipitation
rate would be only a small fraction of that which occurs naturally
(e.g., from volcanoes) without such intervention. However, the
impact of aircraft effluents (via NOx) on ozone, and on the
greenhouse effect itself need to be examined.
An alternative is to pulverize and chemically process in orbital
factories, one small Earth-crossing (Apollo object) or
Earth-approaching (Amor object) asteroid a year. But this is a
voracious waste of the limited number of Apollo or Amor objects, and
much more expensive than delivering an annual layer of transparent
fine particles to stratospheric altitudes from the surface.
The fallout of fine particles from the atmosphere, necessitating a
replacement of the light scattering lay per every year or two, can be
avoided if a continuous solid shield were in place at much higher
altitudes (see, e.g., Fogg 1987).
One proposal to mitigate
greenhouse warming (discussed in Broad 1988) is to deploy a vast
array of orbiting satellites constructed of thin (mylar-like) films
that would attenuate sunlight. However, to compensate for a doubling
in the CO2 abundance, such a satellite array would require an area
equivalent to a few percent of the Earth's surface, if the films
were good reflectors. Such films may be expensive.
At contemporary
prices, 107 km2 of 6 /µm thick aluminized
mylar might cost nearly
$1013; kapton would be perhaps 100 times more expensive (Friedman
1989, personal communication). The progressive darkening of such
films on continued ultraviolet and charged particle irradiation (the
transparency lifetime of mylar films might be about a month) would
be an additional complication.
Conceivably, much cheaper radiation-non-degradable
films might be developed in the future, but they do not appear to be
competitive with some of the alternative possibilities for
ameliorating global warming.
Another proposal (Early 1989) calls for a "glass" shield 2000 km in
diameter and perhaps 10/µm thick, made from lunar materials and
suitably coated, positioned between the Earth and the Sun near the
first Lagrangian point, about 1.5 x 106 km from Earth. The cost is
estimated between $1012 and $1013 and the shield's long-term
radiation and dynamical stability remains to be demonstrated.
Dynamical instabilities may be addressed by equipping the shield
with thrusters. In addition - unlike fine stratospheric particles,
which take about a year to fall out and an orbital particle layer,
which would be extremely difficult to remove - a sunscreen with an
attached propulsion system can quickly be moved if unanticipated
climatic side effects are found to be emerging on Earth.
Better
still for this purpose would be a sunscreen with louvers to
fine-tune its effective opacity. We note also that reflecting
shields need not be at high altitude or in space; massive reflectors
floating in the oceans can also be envisioned, although at unknown
environmental cost.
At best, however, the foregoing class of interventions in the
Earth's climate might be considered as stopgap measures while the
human species improves fossil fuel efficiency, terminates CFC
manufacture, switches to alternative energy sources and takes other
obvious groundbased measures.
But why are any such heroic schemes
needed, as we have already postulated abundant fusion power sometime
in the next century? Why not simply assume that all power generation
will derive from greenhouse-neutral fusion and consider the problem
solved?
The trouble is that even if cheap and safe fusion power were
discovered in the laboratory tomorrow, its development to commercial
scale and its deployment all over the world - including developing
nations that have ready access to fossil fuels (China, for example,
has the second largest coal reserves on Earth) - will take time.
And
in that time, the amount of CO2 continues to build. Moreover, there
are certain troublesome potential feedbacks - e.g., that a few
degrees of global warming will release substantial quantities of
now-sequestered bog methane, further augmenting the greenhouse
effect.
Even if much more serious efforts were made to deal with
global warming than now seem (politically) feasible worldwide, it is
very difficult to imagine stopping the buildup of greenhouse gases
altogether, at least in the next century: the world economy is far
too dependent on fossil fuel energy sources.
Many sources of
greenhouse gases (some 30% of U. S. CO2 emissions derive from
automobiles) are not likely to be replaced by massive fusion power
plants (although electric or hydrogen-fueled autos recharged from
fusion power plants might solve this problem in the long term). So
the consequences of continued greenhouse warming, even if somewhat
delayed, might be considered sufficiently perilous to justify major,
and costly, efforts at amelioration.
However, planetary engineering schemes utilizing extraterrestrial
materials in Earth orbit or at Li, or even terrestrial materials
lofted to stratospheric altitudes, seem to us indeed to be cures
that might be more dangerous than the disease - given both our
present state of ignorance about climatic feedbacks and atmospheric
chemistry, as well as our history of discovering unpleasant
inadvertent side-effects of global scale technology.
An additional
deficit of many such schemes for planetary engineering of the Earth
is that groundbased optical frequency astronomy would essentially
cease. Although most (including us) would consider this an
acceptable sacrifice to preserve the habitability of the Earth, it
would be a tragic loss.
Lagrangian point sunscreens would inhibit
mainly solar astronomy, leaving the rest of the sky no more obscure
than usual.
-
Trees
We advocate instead of particle shields or sunscreens, a well-tried
biological solution, neither endangering astronomy nor posing
unprecedented technological or climatic problems.
We propose
reforesting the world, especially in the tropics, in accordance with
the ancient oriental wisdom,
The added biomass would fix atmospheric CO2, offsetting its
continued buildup.
It would buy time while massive conversion to non-fossil fuel energy sources was underway; it would also have
positive ecological benefits (including relief for many endangered
species); and it would be less likely to produce potentially
disastrous regional shifts in climate. But is reforestation an
allowable solution from the point of view of mass?
The analysis of
this issue was pioneered by Dyson (1977) and Dyson and Marland
(1979). A restatement of the calculation goes as follows: doubling
the CO2 content of the atmosphere from its pre-industrial level
would augment the CO2 abundance by 0.55 g cm-2, or equivalently by
0.15 g cm-2 of C.
The dry biomass in a typical rain forest is about
6 g cm-2 (Lieth and Whittaker 1975), of which about half is
-
Thus,
about 5% of the Earth's surface would need to be reforested with
rain forests (or a higher percentage with other types of forests) to
fix the added CO2 (while leaving stored C in storage).
This is
equivalent to an area of about 2.5 x 107 km2, or about 15% of the
landmass of the Earth. By comparison, tropical rain forests
currently cover somewhat less area, while tropical rain forests,
rain-green forests, and summer-green forests collectively cover a
somewhat larger area than the required 2.5 x 107 km2.
A massive
replanting would be needed, necessarily including tropical latitudes
where forests are now being destroyed on a massive scale for
purposes of short-term commercial profit and "development." Rain
forests serve to stabilize thin layers of topsoil, which,
unfortunately, promptly (after a few growing seasons) erode once the
forest is cut down. This trend towards irreversibility in the
destruction of rain forests makes the practice especially
short-sighted.
Mature forests, on yearly average, fix very little CO2. The desired
steady-state CO2 fixation rate can be achieved with a smaller
fraction of the Earth's surface forested by felling mature forests
and re-planting them with fast-growing timber. This must be done
with care to minimize the penalty to key ecosystems.
As much of this
wood as possible should be used for construction, furniture and
other products of domestic economy.
The remainder should be
sequestered so that it is gradually converted to involatile
kerogens
and, eventually, elemental C. Obviously, burning these trees or the
lumber milled from them defeats the purpose of growing them. When
the world energy economy moves significantly away from fossil fuels,
these strictures could be relaxed.
Net primary production in a tropical rain forest occurs at a rate of
~0.2 g cm-2 yr-1 (Lieth and Whittaker 1975). Thus, ~30 yr is needed
between the initial replanting and the achievement of a steady-state
biomass. This time scale is only somewhat less than current
estimates of the doubling time of atmospheric CO2. It pays to plant
soon.
At their current rate of increase, gases other than CO2 (most
notably CH4, CFCs, and N2O) are generating an additional greenhouse
warming of the atmosphere (Lacis et al. 1981; DOE Multi-Laboratory
Climate Change Committee 1990), which collectively constitute about
50 to 100% of the warming due to added CO2.
Thus, an adequate
strategy for avoiding significant global warming over the next
century must involve controls on these gases as well.
Controls are
already being exercised on CFCs for other environmental reasons. As
cattle and rice paddies are among the primary sources of CH4,
controlling the increase in methane could be integrally tied to such
sensitive issues as the need for animal protein (and other resources
provided by ungulates) and population control in the developing
world, which in any case is a key component of any solution, because
the developing nations together already constitute (after the U. S.
and Russia) the third largest source of CO2 emission on the planet
(Brown 1989).
It has been proposed that the phytoplankton productivity in the
southern ocean is limited by the availability of iron as a
metabolite, that pre-industrial global CO2 abundances were much
higher than would have been the case without this iron deficiency,
and that the abundance of iron-rich atmospheric dust is
anticorrelated with atmospheric CO2 levels (Martin 1990).
This has
led to the suggestion (Martin et al. 1990; Davies 1990) that
fertilizing the southern ocean with 105 to 106 tons of finely
pulverized soluble iron may lead to a phytoplankton bloom that would
significantly mitigate CO2 greenhouse warming. This is the oceanic
equivalent of massive re-forestation and superficially is far easier
- fiscally and politically.
However, unlike re-forestation, this is
an intervention where we have no large-scale experience. More recent
evidence suggests that iron may be much more available to oceanic
phytoplankton than had previously been thought (Wells et al. 1991),
and that ocean dynamic considerations vitiate the idea (Peng and
Broecker 1991).
Even if the iron-deficiency hypothesis were
confirmed (cf. Davies 1990; Joos et al. 1991), it seems wise to
proceed with great caution. Propagating negative ecological
consequences have been suggested; this is also true for alternative
interventions such as massive, periodically harvesting towed seaweed
farms or piping liquefied CO2 to the abyssal depths where, perhaps,
it might solidify (Blakeslee 1990).
Likewise, a scheme for mitigating stratospheric ozone loss by
massive (5 x 104 ton annually) injection of ethane or propane into
the Antarctic stratosphere (Cicerone et al. 1991) is partially
disavowed by the scientists who suggested it on the grounds that it
could increase, not decrease, ozone depletion; one of them is quoted
(Dye 1991) as saying that "the proposal was meant to illustrate that
such a plan is not feasible." (See also Cicerone et al. 1992.)
Since this chapter was prepared, an excellent survey of possible
planetary engineering schemes to mitigate global warming on Earth
has appeared (Committee on Science, Engineering, and Public Policy
1992).
Favored options include re-forestation, delivery of dust to
the stratosphere (using naval gunnery) and increasing oceanic
cloudiness by supplying tropospheric cloud condensation nuclei (via
SO2). Possible side effects - such as enhanced ozone depletion and
acid rain for the latter two schemes - are stressed.
Clearly more
work is needed, but comparatively inexpensive and environmentally
prudent methods of mitigating greenhouse warming on Earth may be
within reach in the next few decades.
VI. SUMMARY
AND RECONSIDERATION OF SOLAR SYSTEM ENVIRONMENTAL ETHICS
We find that with optimistic estimates of late 21st century
technology and physical principles at least moderately
well-understood today, it may be possible to effect massive changes
in the environments and climates of,
...and perhaps
other worlds in the solar system.
Some schemes - such as
impact stripping the atmosphere of Venus or mining much of Mars or
Venus down to depths of hundreds of meters or more - represent a
wholly irresponsible waste of solar system resources and the loss of
irreplaceable scientific knowledge. They are extreme examples of
approaches that must be avoided.
Fortunately, they are also
prohibitively expensive.
Other possible schemes (including those
that utilize the self-replication of genetically engineered
micro-organisms) are less destructive in their immediate impact, but
uncertain in their efficiency and long-term consequences. In
particular, the introduction for a given purpose of microorganisms
into a previously uninhabited planetary environment may, through
evolutionary "adaptive radiation" into untenanted ecological niches,
lead later to a set of entirely unexpected consequences.
Schemes that moderate atmospheric greenhouses by emplacing spherical
shells of fine particles at high altitudes or sunscreens between the
planet and the Sun to some extent avoid both of the preceding
pitfalls:
they are not wholly voracious in their appetite for asteroidal (or cometary) resources, and, because they naturally
decay on a time scale of about a year or can be moved, do not seem
to threaten dangerous long-term consequences.
Because of the high
specific energy of thermonuclear processes, some planetary
engineering schemes that employ fusion power plants in a central
role (e.g., by heating the surfaces of cold worlds) display both
high efficiency and relative environmental responsibility.
It is clear that human technology is now able - even inadvertently,
and certainly were there a concerted and purposeful effort - to
alter entire planetary environments. Doubtless there will be schemes
invented in coming years that are much less energy - and
mass-intensive, much more precise, much freer of inadvertent
side-effects, and much more stable than any proposed here.
We recognize that many people feel a powerful attraction to the idea
of making other worlds in the solar system suitable for human
habitation and then establishing observatories, exploratory bases,
communities, or homesteads there. Because of its pioneering history,
this may be a particularly natural and attractive idea in the United
States.
However, we believe that the motives for altering other
worlds need much more serious consideration than they have received
heretofore.
Because some 240,000 more people are born than die every
day on Earth, emigration to newly engineered worlds cannot be a
feasible means of dealing with the world population crisis. If we
are concerned that the human species might self- destruct, certainly
it makes more sense to devote limited resources to preventing
self-destruction than to preparing an escape hatch for a fortunate
few.
In any case, massive alteration of the environments of other
worlds can be done competently and responsibly only when we have a
much better understanding of those worlds than is available today.
Advocates of planetary engineering should first be advocates of the
long-term and exhaustive scientific exploration of other worlds.
A short-term imperative for planetary engineering exists only for
one world in the solar system, our own.
Careless or reckless
applications of human technological genius have put the global
environment at risk in several different ways. The Earth is not a
disposable planet. It is just conceivable, as we have discussed,
that some of the techniques that in the long term might be applied
to engineering other worlds might also be utilized to ameliorate the
damage being done to this one.
Perhaps a safe way to test our
protocols is to implement them in carefully circumscribed ways on
other worlds. But considering the relative urgencies, a useful
indication of when the human species is ready to consider planetary
engineering seriously is when we have put our own world right. We
can consider it a test of the depth of our understanding and our
commitment.
The first step in engineering the solar system is to
guarantee the habitability of the Earth.
Acknowledgments
This work was supported in part by two
NASA grants.
We thank C. Chyba, F. Dyson, M. Fogg, L. Friedman, J.
Kasting, A. Lacis, C. McKay, G. Marland, J. Oberg, J. Pike, W.
Rossow, R. Turco and, especially, J. Lewis for helpful comments.
REFERENCES
A. C. D. A. 1982. Arms Control and
Disarmament Agreements (Washington, D. C:
U. S. Arms Control and Disarmament Agency). Atreya, S. K.,
Donahue, T. M., and Kuhn, W. R. 1978. Evolution of a nitrogen
atmosphere on Titan. Science 201:611-613. Averner, M. M., and
MacElroy, R. D., eds. 1976. On the Habitability of Mars, NASA
SP-414.
Birch, P. 1991. Terraforming Venus quickly. J. British
Interplanet. Soc. 44:157-167.
Blakeslee, S. 1990. New York Times, Nov. 20, C4.
Broad, W. J. 1988. New York Times, Aug. 16, 1986, CI, C9.
Broecker, W. S. 1985. How to Build a Habitable Planet
(Palisades, N. Y: Eldigio Press).
Brown, L. 1989. The State of the World, 1989 (New York: W. W.
Norton). Budyko, M. I. 1974. Meteorology and Hydrology 2:91-97
(in Russian). Budyko, M. I. 1977. Climatic Changes (Washington,
D. C: American Geophysical Union).
Burns, J. A., and Harwit, M. 1973. Towards a more habitable Mars
- or - the coming
Martian spring. Icarus 19:126-130. Chamberlain, J. W., and
Hunten, D. M. 1987. Theory of Planetary Atmospheres (San
Diego: Academic Press), esp. pp. 136-140. Chaun, R. L., and
Woods, D. C. 1984. Comets, ice ages, and ecological
catastrophes.
Geophys. Res. Lett. 11:553-556. Cheney, R. B. 1989. Soviet
Military Power: Prospects for Change (Washington,
D. C: U. S. Dept. of Defense). Cicerone, R. J., Elliott, S., and
Turco, R. P. 1991. Reduced antarctic ozone depletions
in a model with hydrocarbon injections. Science 254:1191-1194.
Cicerone, R. J., Elliot, S., and Turco, R. P. 1992. Global
environmental engineering.
Nature 356:472.
Committee on Science, Engineering, and Public Policy. Panel on
Policy Implications of Greenhouse Warming. 1992. Policy
Implications of Greenhouse Warming: Mitigation, Adaptation, and
the Science Base (Washington: National Academy Press).
Cordey, J. G., Goldston, R. J., and Parker, R. R. 1992. Progress
toward Tokamak
fusion. Physics Today 45:22-30. Davies, A. G. 1990. Taking a
cool look at iron. Nature 345:114-115.
DOE Multi-Laboratory Climate Change Committee. 1990. Energy and
Climate
Change (Chelsea, Mich.: Lewis Publishers). Dye, L. 1991. Los
Angeles Times, Dec. 10. Dyson, F. J. 1977. Energy 2:287-291.
Dyson, F. J. 1989. Terraforming Venus [letter/response to M. J.
Fogg, The terraforming of Venus, 40:551-564]. /. British
Interplanet. Soc. 42:593.
Dyson, F. J., and Marland, G. 1979. In Carbon Dioxide Effects
Research and Assessment Program: Workshop on the Global Effects
of Carbon Dioxide from Fossil Fuels, eds. W. P. Elliott and L.
Machta, March 7-11, Miami Beach, Fl. U. S. Dept. of Energy,
Conf. Rept. 770385, pp. 111-118.
Early, J. T. 1989. Space-based solar shield to offset greenhouse
effect. /. British Interplanet. Soc. 42:567-569.
Fogg, M. J. 1987. Space-based solar shield to offset greenhouse
effect. J. British Interplanet. Soc. 40:551-564.
Fogg, M. J., ed. 1989. Terraforming. J. British Interplanet.
Soc. Special Issue 42:553.
Fogg, M. J. 1992. A synergic approach to terraforming Mars. /.
British Interplanet. Soc. 45:315-329.
Gillett, S. L. 1991. Establishment and stabilization of
Earthlike conditions on Venus. /. British Interplanet. Soc.
44:151-156.
Hansen, J., Fung, I., Lacis, A., Rind, D., Lebedeff, S., Ruedy,
R., Russell, G, and Stone, P. 1988. Global climate changes as
forecast by Goddard Institute for Space Studies
Three-Dimensional Model. /. Geophys. Res. 93:9341-9364.
Harwell, M. A., and Hutchinson, T. C. 1985. Environmental
Consequences of Nuclear War: Volume II. Ecological and
Agricultural Effects (New York: J. Wiley).
Hoyle, F., and Wickramasinghe, N. C. 1978. Comets, ice ages, and
ecological catastrophes. Astrophys. Space Sci. 53:523.
Joos, F., Sarmiento, J. L., and Siegenthaler, U. 1991. Estimates
of the effects of South Ocean iron fertilization on atmospheric
CO2 concentrations. Nature 349:772-775.
Lacis, A., Hansen, J., Lee, P., Mitchell, T., and Lebedeff, S.
1981. Greenhouse effect of trace gases, 1970-1980. Geophys. Res.
Lett. 8:1035-1038.
Latham, J. 1990. Control of global warming. Nature 347:339-340.
Lieth, H., and Whittaker, R. H. 1975. Primary Productivity in
the Biosphere (New York: Springer-Verlag).
Lovelock, J., and Allaby, M. 1985. The Greening of Mars (New
York: St. Martins Press).
Martin, J. H. 1990. Glacial-interglacial CO2 change: The iron
hypothesis. Paleocean. 5(1):1-13.
Martin, J. H., Gordon, R. M., and Fitzwater, S. E. 1990. Iron in
antarctic wars. Nature 345:156-158.
McKay, C. P., Pollack, J. B., and Courtin, R. 1989. The thermal
structure of Titan's
atmosphere. Icarus 80:23-53. McKay, C. P., Toon, O. B., and
Kasting, J. F. 1991. Making Mars habitable. Nature
352:489-496.
Melosh, H. J., and Vickery, A. M. 1989. Impact erosion of the
primordial Martian atmosphere. Nature 338:487^89.
Morrison, D. 1992. The Spaceguard Survey: Report of the NASA
International Near-Earth-Object Detection Workshop, ed. D.
Morrison (Pasadena: Jet Propulsion Laboratory).
NASA. 1988. Present State of Knowledge of the Upper Atmosphere:
An Assessment Report (Washington, D. C: U. S. Government
Printing Office).
NIOSH/OSHA. 1978. Pocket Guide to Chemical Hazards (Washington,
D. C: U. S. Depts. of HEW and Labor).
Oberg, J. 1981. New Earths (Harrisburg, Perm.: Stackpole Books).
Peng, T.-H., and Broecker, W. S. 1991. Dynamical limitations on
the antarctic iron
fertilization strategy. Nature 349:227-229. Pittock, A. B.,
Ackerman, T. P., Crutzen, P. J., MacCracken, M. C, Shapiro, C.
S.,
and Turco, R. P. 1986. Environmental Consequences of Nuclear
War: Volume I.
Physical and Atmospheric Effects (New York: J. Wiley). Pollack,
J. B. 1979. Climate change on the terrestrial planets. Icarus
37:479-553. Pollack, J. B. 1991. Kuiper Prize Lecture: Present
and past climates of the terrestrial
planets. Icarus 91:173-198. Pollack, J. B., Toon, O. B., and
Boese, R. W. 1980. Greenhouse models of Venus' high
surface temperature, as constrained by Pioneer Venus
measurement. J. Geophys.
Res. 85:8223-8231.
Pollack, J. B., Kasting, J. E, Richardson, S. M., and Poliakoff,
K. 1987. The case for
a wet, warm climate on early Mars. Icarus 71:203-224. Pollack,
J. B., Roush, T., Whitteborn, E, Bregman, J., Wooden, D.,
Stoker, C, Toon,
O. B., Rank, D., Dalton, B., and Freedman, R. 1990. Thermal
emission spectra
of Mars (5.4 - 10.5 fim): Evidence for sulfates, carbonates, and
hydrates. J.
Geophys. Res. 95:14595-14628. Polo, M. C. 1300. // Milione.
English trans. The Travels of Marco Polo, ed. R. E.
Latham (Penguin, 1958), p. 156. Ryan, J. A. 1964. Notes on the
Martian yellow clouds. J. Geophys. Res. 69:3759-
3770.
Sagan, C. 1960. The Radiation Balance of Venus. JPL Tech. Rept.
32-34 (Pasadena:
Jet Propulsion Lab). Sagan, C. 1961. The planet Venus. Science
133:849-858.
Sagan, C. 1973. Liquid carbon dioxide and Martian polar laminas.
/. Geophys. Res. 78:4250-4251.
Sagan, C. 1986. Honda Prize Address. Transcript available from
The Honda Foundation, Tokyo.
Sagan, C. 1992. Between enemies. Bull. Atomic Scientists
48:24-26.
Sagan, C. A., and Mullen, G. 1972. Earth and Mars: Evolution of
atmospheres and
surface temperatures. Science 177:52-56. Sagan, C, and Turco, R.
1990. A Path Where No Man Thought: Nuclear Winter and
the End of the Arms Race (New York: Random House). Seckbach, J.,
and Libby, W. F. 1969. Vegetative life on Venus? Or
investigations with
algae which grow under pure CO2 in hot acid media and at
elevated pressures. In
Planetary Atmospheres, eds. C. Sagan, T. C. Owen and H. J. Smith
(Dordrecht:
D. Reidel), pp. 62-83. Sittig, M. 1985. Handbook of Toxic and
Hazardous Chemicals, 2nd ed. (New York:
Noyes).
Stapledon, O. 1948. Interplanetary man? /. Brit. Interplanet.
Soc. 7:213-233.
Strong, A. E. 1989. Greater global warming revealed by
satellite-derived sea-surface-temperature trends. Nature
338:642-645.
Turco, R. P., Toon, O. B., Ackerman, T. P., Pollack, J. B., and
Sagan, C. 1983. Nuclear winter: Global consequences of multiple
nuclear explosions. Science 222:1283-1292.
Turco, R. P., Toon, O. B., Ackerman, T. P., Pollack, J. B., and
Sagan, C. 1990. Climate and smoke: An appraisal of nuclear
winter. Science 247:166-176.
Vickery, A. M., and Melosh, H. J. 1990. Atmospheric erosion and
impactor retention in large impacts, with application to mass
extinction. In Global Catastrophes in Earth History, eds. V. L.
Sharpton and P. D. Ward, Geological Soc. of America SP-247, pp.
289-300.
Walker, J. C. G. 1986. Impact erosion of planetary atmospheres.
Icarus 68:87-98.
Wells, M. L., Mayer, L. M., Donard, O. F. X., de Souza Sierra,
M. M., and Ackelson, S. G. 1991. The photolysis of colloidal
iron in the oceans. Nature 353:248-250. Wittenberg, L. J.,
Santarius, J. F., and Kulcinski, G. L. 1986. Fusion Tech.
10:167-178. WMO. 1985. Report 16 (Geneva: World Meteorological
Organization).
|