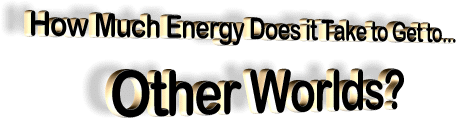
by Matt Williams
July 21, 2016
from
UniverseToday Website
PDF format
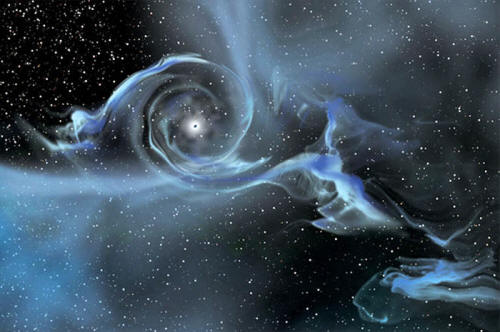
Traveling to another body in our Solar System requires an immense
amount of planning, preparation, and resources.
When plotting the
Journey to
the Moon in the 1960s, NASA committed to years of
research and development, studying not only spacecraft designs, but
also mission profiles. All of this culminated in the creation of all
the necessary components that led to the historic Moon Landing on
July 20th, 1969.
With our eyes now fixed
on Mars and other planets
in the Solar System, similar planning has to be made.
When plotting a journey to Earth's only satellite - which lies at an
average distance of 384,399 km (238854.5 mi) from Earth - required a
huge commitment in resources and energy. Mars, on the other hand,
made its closest approach to Earth in 2003, at a distance of 56
million km (34.8 million mi).
Because of this, getting there will be
much more taxing.
So the question is, when looking to Mars and
beyond, how much energy will it take for a spacecraft to make the
journey?
Potential
Targets
As mentioned already, Mars and Earth were at their closest in 2003.
But due to the nature of our orbits around the Sun - Earth's being
interior to Mars, and Mars having an eccentric orbit around the Sun
- this distance varies considerably. The two planets make their
closest approach - what is called "opposition" - every 26 months,
when Mars is at perihelion (closest to the Sun) and Earth is at
aphelion (farthest).
At this time, they can be as close as 55 million km, which is why
space agencies try to take advantage of this window to launch
missions to the Red Planet.
On the other side of things, Earth and
Mars are at their greatest distance from each other when their
positions are reversed - Earth being at perihelion and Mars at
aphelion.
At this point, which described as the planets being at
"conjunction", Earth and Mars are a distance of 225 million km (140
million mi).
However, Mars is not Earth's closest neighbor. That honor goes to
Earth's "Sister Planet", Venus, which lies at a distance of 41
million km (25.5 million mi) when it is at its closest.
This happens
when Venus orbits between Earth and the Sun - what is an inferior
conjunction - which occurs every 584 days (1.6 years).
When Venus
and Earth are on opposite sides of the Sun - a superior conjunction
- they are nearly 257 million km (159.7 million mi) apart. Because
of this, Venus would not only take less time and energy to travel
to, but the launch windows would occur more often.
Mercury is similarly located between earth's orbit and the Sun,
which means its distance varies between it being at an inferior and
a superior conjunction. At an inferior conjunction, Earth and
Mercury are an average of km 77.3 million km (48 million mi).
When
it is at superior conjunction, it is 221.9 million km (137.88
million mi). Mercury is at an inferior conjunction to Earth every
116 days, or approximately once every four months.
Next up, Jupiter, king of the planets!
As an planet of the outer
Solar System, its distance from Earth is significantly greater than
any of the inner Solar plants. Case in point, when Jupiter and Earth
are at their closest (opposition), Jupiter is at an average distance
of 588.5 million km (365.68 million mi) from us.
That's over twice as far as Mercury and Mars are at their most
distant. And when it is at its most distant (conjunction), it is
968.1 million km (601.55 million mi). However, despite its distance
and its rather wide orbit around the Sun, it only takes about 399
days (1.09 years) for it to experience a conjunction with Earth.
This would allow for a decent launch window, provided a ship can get
their speedily to catch Jupiter before it moves too far away.
Saturn, another gas giant prominently occupying the outer Solar
System, takes slightly less than a year to experience a conjunction
with Earth. At its closest, it is 1195.5 million km (742.85 million
mi) from Earth; and at its most distant, it is 1.658 billion km
(1.03 billion mi) distant.
Again, a relatively good launch window,
but the distances involved complicate things.
When we get to the gas/ice giants, things become even more
interesting. At its closest to Earth, Uranus is an average of 2.58
billion km (1.604 billion mi) distant, which only occurs once every
few decades. At its greatest distance, Uranus is 3.157 billion km
(1.96 billion mi) from Earth.
Neptune, the most distant of the planets, is a whopping 4.3 billion
km (2.67 mi) from Earth when it is at opposition, which takes 367.5
days on average.
When it is at conjunction, that distance does not
vary significantly, reaching a distance of 4.7 billion km (29.2
billion mi) from Earth.
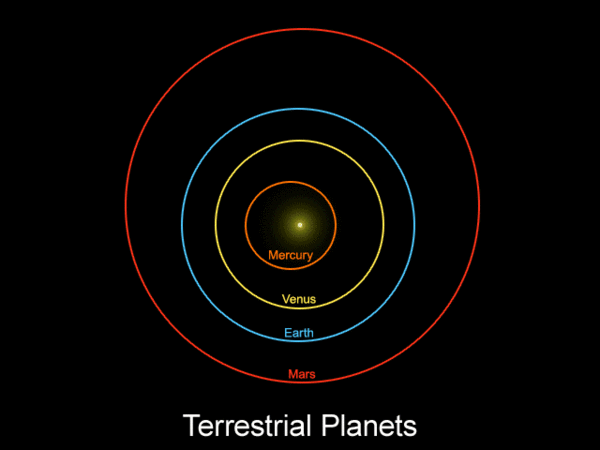
Animated diagram
showing the spacing of the Solar Systems planet's,
the unusually closely
spaced orbits of six
of the most distant
KBOs, and the possible “Planet 9”.
Credit: Caltech/nagualdesign
To break these numbers down, no planet
is ever at the same distance from Earth at any given time.
The fact
that they are also revolving around our Sun means their distance
from us varies considerably, which in turn means we cannot travel to
them in a straight line.
Hence why missions to distant planets often
have to take indirect routes, and rely on "gravity assists" from
other planets.
Potential
Methods
Thanks to decades of space exploration, we have a pretty good
framework for determining how much energy it would take to get to
neighboring planets.
Essentially, we have sent missions to every
planet that orbits the Sun, and even some of the dwarf planets and
moons. So determining how much energy would be involved using
current methods involves very little guess-work.
For the record, when we talk about potential methods, we are talking
about those that either rely on existing technology, or those that
do not yet exist, but are technically feasible. In just about all
cases, they present a possible, but time-consuming means of getting
to another planet.
How much energy they will consume in the
process, that depends on the method:
Conventional Rockets
This form of propulsion, which is the most time-honored and widely
used, involves igniting solid or liquid chemical propellants, and
then channeling the resulting combustion through nozzles to generate
thrust.
It was this very technology that powered the Saturn V
rockets and the spacecraft that were used by
the Apollo Program to
put astronauts on the Moon also relied on a combination of chemical
and liquid propellants.
For instance, the first stage of the Saturn V used a combination of
refined kerosene and liquid oxygen, while its upper stages used
liquid hydrogen.
The Command/Service and Apollo Modules - which made
the flight to the Moon and landed the crew on the surface - relied
on a series of jets that used a combination of chemical propellant
and oxidizer, as well as stored helium to pressurize them.
Using
this technology, the Apollo 11 mission took about three days,
launching on July 16th and achieving orbital insertion on July 19th,
1969.
A more contemporary example of this would be the engine system on
the
New Horizons
mission - which consisted of 16 thrusters fueled with hydrazine
monopropellant.
Using this technology, New Horizons
reached the Moon
in a mere 8 hours and 35 minutes before heading off towards Pluto.
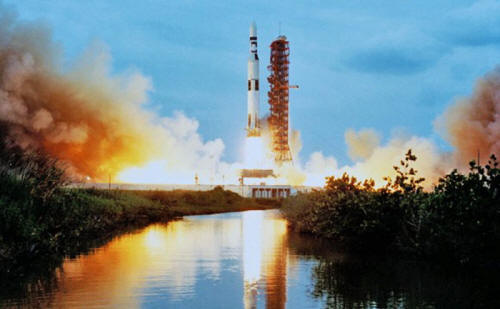
A Saturn V rocket
launch,
carrying the Skylab
space station into orbit in 1973.
Credit: NASA
The New Horizons probe also passed the orbit of Mars on April 7th,
2006 (79 days after launch) while the probe was moving at a speed of
roughly 21 km/s (76,000 km/h; 47,000 mph).
It then reached the
Jovian system in 2007, where it conducted a close flyby to pick up a
gravity boost on its way to Pluto. It's closest pass to Jupiter
happened on February 28th, 2007 - roughly 1 year, 5 weeks and 5 days
(405 days) after launch.
Similarly, the Voyager 1 mission launched from earth on September
5th, 1977 and arrived around Jupiter on March 5th, 1979.
All told,
it took the spacecraft 1 year and 26 weeks (547 days) to make the
trip. Voyager 2 launched a few months later. on August 20th, 1977,
and arrived around Jupiter on July 9th, 1979 - 1 year, 10 months,
and 19 days (688 days).
Ionic
Propulsion
Currently, ionic propulsion is the
slowest form of propulsion, but also the most fuel-efficient.
And
its only been in recent years that the technology to support ion
engines has moved from theory to practice. A good example of this is
the ESA's
SMART-1 mission,
which successfully completed its mission to the Moon after taking a
13 month spiral path from the Earth.
SMART-1 used solar powered ion
thrusters, where electrical energy is harvested from its solar
panels and used to power its
Hall-effect thrusters.
Only 82 kg of xenon propellant was used to propel SMART-1 to the
Moon. 1 kg of xenon propellant provided a delta-v of 45 m/s.
This is
a highly efficient form of propulsion, but it is by no means fast.
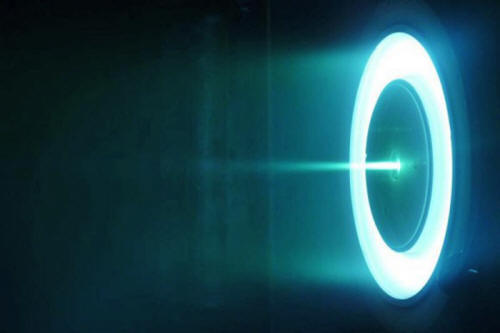
Ionic propulsion is
currently the slowest,
but most
fuel-efficient, form of space travel.
Credit: NASA/JPL
One of the first missions to use ion
drive technology was the
Deep
Space 1 mission to Comet
Borrelly that took place
in 1998.
DS1 also used a xenon-powered ion drive, consuming 81.5 kg
of propellant. Over 20 months of thrusting, DS1 was managed to reach
a velocity of 56,000 km/hr (35,000 miles/hr) during its flyby of the
comet.
And then there was NASA's
Dawn
mission, which relied on
an ion engine similar to the SMART-1 mission to reach the Asteroid
Belt. After launching from Earth on September 27th, 2007, the probe
achieved an orbit around Ceres on March 6th, 2015 - 7 years 25 weeks
(2555 days) later.
Ion thrusters are more economical than rockets, as
the thrust per unit mass of propellant (a.k.a. specific impulse) is
far higher.
But it takes a long time for ion thrusters to accelerate
spacecraft to any great speeds, and the maximum velocity it can
achieve is dependent on its fuel supply and how much electrical
energy it can generate.
Gravity Assist Method
The fastest existing means of space travel is known
the Gravity Assist method, which involves a spacecraft using the
relative movement (i.e. orbit) and gravity of a planet to alter is
path and speed.
Gravitational assists are a very useful spaceflight
technique, especially when using the Earth or another massive planet
(like a gas giant) for a boost in velocity.
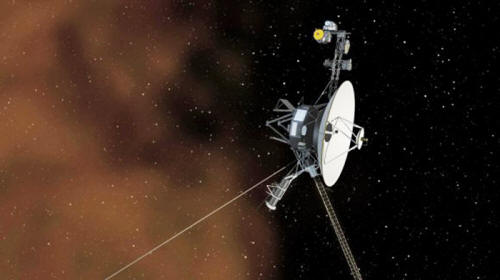
Artist's concept of
Voyager 1 in interstellar space.
Credit:
NASA/JPL-Caltech
The
Mariner 10
spacecraft was the first to use this method, using Venus'
gravitational pull to slingshot it towards Mercury in February of
1974.
In the 1980s, the
Voyager 1 probe used
Saturn and Jupiter for gravitational slingshots to attain its
current velocity of 60,000 km/hr (38,000 miles/hr) and make it into
interstellar space.
However, it was the
Helios
2 mission - which was
launched in 1976 to study the interplanetary medium from 0.3 AU to 1
AU to the Sun - that holds the record for highest speed achieved
with a gravity assist.
At the time,
Helios 1 (which launched in 1974) and
Helios 2 held the record for
closest approach to the Sun. Helios 2
was launched by a conventional NASA Titan/Centaur launch vehicle and
placed in a highly elliptical orbit.
Due to the large eccentricity (0.54) of the 190 day
solar orbit, at perihelion Helios 2
was able to reach a maximum velocity of over 240,000 km/hr (150,000
miles/hr). This orbital speed was attained by the gravitational pull
of the Sun alone.
Technically, the Helios 2 perihelion velocity was not a gravitational slingshot,
it was a maximum orbital velocity, but it still holds the record for
being the fastest man-made object regardless.
Nuclear Thermal and Nuclear
Electric Propulsion (NTP/NEP)
Another possibility for interstellar
space flight is to use spacecraft equipped with
nuclear engines,
a concept which NASA has been exploring for decades.
In a Nuclear
Thermal Propulsion (NTP) rocket, uranium or deuterium reactions are
used to heat liquid hydrogen inside a reactor, turning it into
ionized hydrogen gas (plasma), which is then channeled through a
rocket nozzle to generate thrust.
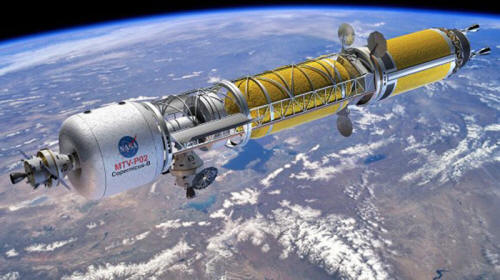
Artist's concept of a
Bimodal Nuclear
Thermal Rocket in Low Earth Orbit.
Credit: NASA
A Nuclear Electric Propulsion (NEP) rocket involves the same basic
reactor converting its heat and energy into electrical energy, which
would then power an electrical engine.
In both cases, the rocket
would rely on nuclear fission or fusion to generates propulsion
rather than chemical propellants, which has been the mainstay of
NASA and all other space agencies to date.
Compared to chemical propulsion, both NTP and NEC offers a number of
advantages. The first and most obvious is the virtually unlimited
energy density it offers compared to rocket fuel. In addition, a
nuclear-powered engine could also provide superior thrust relative
to the amount of propellant used.
This would cut the total amount of
propellant needed, thus cutting launch weight and the cost of
individual missions.
Although no nuclear-thermal engines
have ever flown, several design concepts have been built and tested
over the past few decades, and numerous concepts have been proposed.
These have ranged from the traditional solid-core design - such as
the
Nuclear Engine for Rocket Vehicle
Application (NERVA) - to
more advanced and efficient concepts that rely on either a liquid or
a gas core.
However, despite these
advantages in fuel-efficiency and specific impulse, the most
sophisticated NTP concept has a maximum specific impulse of 5000
seconds (50 kN·s/kg).
Using nuclear engines driven by fission or
fusion, NASA scientists estimate it would could take a spaceship
only
90 days to get to Mars
when the planet was at “opposition” - i.e. as close as 55,000,000 km
from Earth.
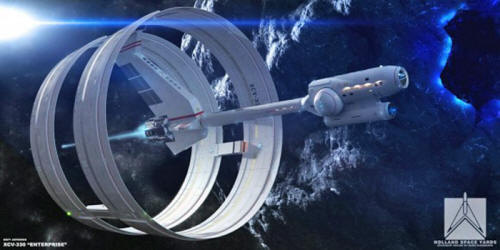
Artist's concept of
an
interstellar craft
equipped with an EM Drive.
Credit: NASA
Spaceflight Center
Electromagnetic (EM) Drive
Another possible method of travel
comes in the form of the Radio Frequency (RF) Resonant Cavity
Thruster, also known as the EM Drive.
Originally proposed in 2001 by
Roger K. Shawyer, a UK scientist who started
Satellite Propulsion Research Ltd
(SPR) to bring it to fruition, this drive is built around the idea
that electromagnetic microwave cavities can allow for the direct
conversion of electrical energy to thrust.
Whereas conventional EM thrusters are designed to
propel a certain type of mass (such as ionized particles), this
particular drive system relies on no reaction mass and emits no
directional radiation.
Such a proposal has met with a great deal of
skepticism, mainly because it violates the law of Conservation of
Momentum - which states that within a system, the amount of momentum
remains constant and is neither created nor destroyed, but only
changes through the action of forces.
However, experiments conducted in the past few years
have apparently yielded positive results.
Based on calculations
performed using the NASA prototype, which yielded a power estimate
of 0.4 N/kilowatt, a spacecraft equipped with the EM drive could
make the trip to Pluto in less than 18 months (126 days). That's
one-sixth the time it took for the New Horizons probe to get there,
which was traveling at speeds of close to 58,000 km/h (36,000 mph).
Naturally, further experimentation is needed to confirm that this is
truly possible.
***
As you can see, there are a few means of getting
around the Solar System, ranging from the gas-guzzling but rapid, to
the slow and steady. In all cases though, it comes down to a massive
expenditure of energy.
While some are all about using it quickly to
achieve high acceleration, others apply the energy in small amounts
over a long period of time.
Other means are still on the shelf, but could very
well be assembled using today's technology. These (ranging from the
EM Engine to Nuclear power) emphasize tackling the problem with
increasingly sophisticated forms of technology.
And while they do
offer shorter trip times and a more energy-efficient mode of travel,
some serious development needs to happen before they can be
considered readily available.
|