
by Robert A. Freitas
1976
Updated 2008
from
Xenology Website
"Who knows for certain? Who
shall declare it?
Whence was it born, whence came creation?
The gods are later than this world’s formation;
Who then can know the origins of the world?
None knows whence creation arose;
And whether he has or has not made it;
He who surveys it from the lofty skies,
Only he knows - or perhaps he knows not."
-- Rig Veda, ca. 1000
B.C.
"If a dirty undergarment is squeezed into the mouth of a
vessel containing wheat within a few days (say 21) a ferment
drained from the garments and transformed by the smell of
the grain, encrusts the wheat itself with its skin and turns
it into mice. And what is more remarkable, the mice from
corn and undergarments are neither weanlings nor sucklings
nor premature, but they jump out fully formed."
-- Jan Baptista van
Helmont (1577-1644)
"It is often said that all the conditions for the first
production of a living organism are now present, which could
have been present. But if (and oh! what a big if!) we could
conceive in some warm little pond, with all sorts of ammonia
and phosphoric salts, light, heat, electricity, etc.,
present, that a proteine compound was chemically formed
ready to undergo still more complex changes, at the present
day such matter would be instantly devoured or absorbed,
which would not have been the case before living creatures
were formed."
-- Charles Robert
Darwin (1809-1882)
"Ultimately, such a scientist is saying that man’s mind was
created by a batch of dancing chemicals. He is saying that
Shakespeare and St. Francis of Assisi were manufactured by
something like Alka-Seltzer fizzing in a glass."
-- in The Sign, a
Catholic monthly (1956)
"The molecules that could not copy themselves did not. Those
that could, did. The number of copying molecules greatly
increased..."
-- Carl Sagan, in The
Cosmic Connection (1973)
Scientists today will still admit that
they really don’t know how life began on our planet.
Laboratory work is tricky, and nobody
was present to witness events at first hand on the primitive Earth.
Researchers in abiogenesis can only invent some reasonable story
about how life arose, and then maximize its plausibility by
theoretical and experimental investigations.
There are two central themes that run as undercurrents throughout
the whole of xenobiology. First, what is the probability that life
of our kind will evolve on other worlds? By illuminating the
abiogenic processes of this planet in ancient times, scientists hope
to get a handle on the exact combination of conditions and events
necessary for the origin of carbon-based Earthlike life anywhere in
the Galaxy.
The second central theme of xenobiology, to which we shall return in
later chapters, is the likelihood that life, once having emerged in
a planetary environment, will constitute a form of biota more or
less similar to that found on Earth. The laws of biochemistry demand
that molecules combine only in certain specific ways, and usually
only in a very few most probable ways.
In other words, what are the physical
and biochemical limits of the possible?
Historical Views on
the Origin of Life
Speculations on the source of life have been abundant throughout
recorded history. The Rig Veda mentions that biology began from the
primary elements, and the Atharva Veda suggests that the oceans were
the cradle of life. The Bible, with its contradictory accounts of
the Creation in Genesis (did man arrive before or after the
beasts?), is strictly adhered to by many fundamentalists.
Philip Henry Gosse, an eminent 19th century zoologist and Christian,
found it a simple task to reconcile the growing mass of paleontological evidence with the Scriptures. God, he declared,
created the Earth entirely in accordance with scientific findings.
The Lord fabricated geological strata, embedded fossils and the like
for the sole purpose of fooling geologists.
The apparently extreme
age of Earth is only an illusion.
Peculiar ideas abound. Hylozoism, for instance, is the belief that
matter and life are one and inseparable. From this viewpoint, life
either has no origin and has always existed, or else the question
may be deferred to the origin of all matter.
The theory of pyrozoa, to cite another example, was advanced by
William Preyer in the last century.
Preyer believed that life has
existed at all times, even when our planet was still in the molten
state. These first fiery living things, the pyrozoa, slowly modified
and adapted themselves as the environment cooled and changed,
eventually assuming the form in which life presents itself to us
today.
Most theories on the origin of life have fallen into one of four
distinct categories:
-
Life has no origin - both life
and matter have existed forever
-
Life is the consequence of a
supernatural event, intractable and in explicable by the
methods of science
-
Life originated via ordinary
chemical evolution in a deterministic fashion - under
similar circumstances, the same general evolutionary
patterns would repeat themselves on any world
-
Life originated elsewhere by
means unknown, and was subsequently transported to Earth (panspermia).
The first two are self-explanatory, and
the third closely approximates the leading modern theories. The last
deserves a word of explanation.
The Greek philosopher Anaxagoras of Clazomenae (ca. 500-428 B.C.)
was possibly the first to suggest that the seeds of life permeated
the universe. With the downfall of spontaneous generation millennia
later, panspermia enjoyed a brief revival. The theory was sponsored
by many 19th-century notables, including Richter, Kelvin, Helmholtz,
Arrhenius, and the great Italian chemist Avogadro.
The doctrine of lithopanspermia held that meteorites were the means
by which life wandered from planet to planet throughout the cosmos.
Lord Kelvin, a central proponent of this view, considered it
probable that countless life-bearing "stones" existed in space,
perhaps as the result of collisions between inhabited worlds.
Hermann von Helmholtz, a German
philosopher and a pioneer in physics, believed that the interior of
a meteorite would be a safe retreat for interplanetary microbes
during the long incandescent journey through thick planetary
atmospheres. The presence of hydrocarbons in the carbonaceous
chondrites was cited as evidence of the biological activities of the
tiny organisms from space.
Modern analyses suggest that microscopic lifeforms embedded in
interstellar comets are possible, but unlikely. The accumulated
radiation dose from cosmic rays and natural internal radioactivity
is "embarrassingly high" over the large transit times involved
between worlds.
Furthermore, it is now known that
meteorites are of roughly the same age as the rest of the solar
system, and that the organic molecules found in chondrites are
reproducible by strictly chemical means.
The famous Swedish physical chemist and
Nobelist Svante Arrhenius was the loudest advocate for the theory of
radiopanspermia.2304,2305,2306 He suggested that minute spores might
be carried upward through planetary atmospheres by convection, where
electrical forces could provide sufficient energy to expel them from
the body.
The pressure of sunlight would then be
enough to propel these cosmozoa to other solar systems. Tramping
through space, or riding piggyback on small grains of dust, these
legions of microscopic interstellar emissaries thus brought the good
news of life to the rest of the Galaxy.
Carl Sagan has done a careful analysis of the problem, the details
of which will not be repeated here. His conclusion is that
radiopanspermia is not a viable theory of the origin of life on
Earth. Those microbes ejected from a stellar system by radiation
pressure accumulate a dose of x-rays and UV three or four orders of
magnitude higher than the maximum lethal irradiation sustain able by
even the hardiest terrestrial organisms.
Shielding won’t help:
Life-forms large enough not to be killed aren’t ejected by radiation
pressure because they are too heavy.
The theory that life arose in the ancient swirling gas and dust
clouds of interstellar space and then traversed the cosmos, seeding
the Galaxy with life, may be called cosmospermia.
Dr. J. Mayo Greenberg at New York
State University set up a laboratory experiment a few years ago,
using tiny grains of matter the size of space dust and appropriate
gases. He found that many compounds of relatively high molecular
weight could be formed under the influence of ultraviolet radiation.
Greenberg evidently believes that a similar mechanism could lead to
the production of grains of a size and composition similar to that
of viruses.
Dr.
Sagan has disputed such theories, noting that any hypothetical
extraterrestrial organism of 10-5 cm - the size of a rabies virus or
the PPLO (the smallest lifeform known) - would have a replication
time on the order of two hundred million years. There could only
have been fifty or so generations since the Galaxy first formed,
insufficient time for natural selection and evolution to operate.
It is hard to imagine a smaller yet
viable organism; the replication time for a larger microbe would be
even longer, permitting still fewer generations.
Accidental panspermia is a class of theory typified by the "Gold
Garbage Theory," popularized by Dr. Thomas Gold, a leading
astrophysicist at the Center for Radiophysics and Space Research at
Cornell University.
The
Garbage Theory was first
announced in a paper read before a Los Angeles meeting of space
scientists in late 1958, and proposes that Earth may have been
visited by an expedition of advanced ETs who carelessly allowed some
of their native microbiota (picnic basket litter?) to escape.
"While this garbage theory of the
origin of life understandably lacks appeal," one xenologist
notes wryly, "we should not exclude it altogether."
A similar idea is the concept of
directed
panspermia, which suggests that organisms were deliberately
transmitted to Earth by intelligent beings on another planet.
Advanced civilizations might intentionally seed sterile worlds,
either as a prelude to colonization or perhaps simply to perpetuate
the heritage of life on the home planet as insurance against
catastrophe.
Panspermia does not address the phenomenon of
abiogenesis but merely
displaces the problem in space and time.*
Consequently, panspermia hypotheses
aren’t strictly relevant to the ultimate origin of life in the
universe but simply explain how any particular world might have come
to be inhabited.
* One science fiction
story suggests that life on Earth may have arisen from biota left
behind by a careless time traveler from our planet’s future.636 If
any theory begs the question it is this one!
Cosmochemical
Evolution
The building blocks for life are lying around everywhere.
Great clouds of organic molecules have been discovered drifting
between the stars, presumably formed by various natural processes.
Radioastronomers have seen relatively complex compounds hiding deep
in inter stellar space, including methyl alcohol, ethyl alcohol,
cyanogen, formaldehyde, formic acid and ether, and the search is on
for amino acids.
Compounds of carbon and hydrogen, particularly cyanogen, methane and
hydrocarbon radicals, are detected on the surfaces of
stars.1973,2297 To find the limits of such processes, Dr. John Oró
performed an experiment which simulated a hot stellar plasma. Using
a graphite resistance apparatus and a plasma torch device
temperatures from 1500-4000°C were obtained.
Methane, ammonia and water were
introduced continuously. The products were condensed at room
temperature and allowed to interact for a few hours before analysis.
Three amino acids appeared - alanine, glycine, and aspartic acid -
along with hydrogen cyanide and a host of other organics.
There is no doubt that the carbon compounds essential for the
development of Earthly life are ubiquitous. Organics have been
detected on the Moon, other planets, asteroids, and in comets.
The carbon chemistry of meteorites is also well-documented.
The Murcheson rock which fell in Australia on September 28, 1969
contains 2×10-7 moles of amino acids per gram of
meteorite, which is more than many desert sands on Earth. These
amino acids correspond rather closely to those produced in prebiotic
synthesis experiments performed in the laboratory.
The Orgueil meteorite contains
approximately 7% organic matter, including hydrocarbons, fatty
acids, aromatics, porphyrins, nucleic acid bases, optically active
lipids, and a variety of polymeric material. On the basis of the
amounts of carbon compounds detected in various meteorites,
researchers have concluded that these interplanetary wanderers could
have brought as much as 5×1010 kg of formaldehyde and
3×1011 kg of amino acids to Earth during the first eon of
its existence.
Taken together, these studies of meteorites, comets, planets and
interstellar matter strongly suggest that chemical evolution is a
continuing and commonplace occurrence in all parts of the cosmos.
The basic constituents necessary for the emergence of life are
universal.
This implies that life should be widely
distributed throughout the Galaxy, wherever conditions are clement,
since the required ingredients of abiogenic processes are abundantly
available everywhere.
Early Chemical
Evolution on Earth
Chemical evolution refers to the period in Earth’s history during
which the chemical components on the surface changed from simple
forms into complex substances from which the first living organisms
- protobionts - could develop. The primary investigative tool in
abiogenesis research has been the prebiotic synthesis experiment.
Plausible primitive Earth conditions are
arranged in a closed laboratory apparatus, and the changes that take
place are carefully monitored.
The argument has long been made that since no geological record of
the origin of life exists, the course of events leading up to the
creative event is fundamentally unknowable. While most biochemists
today would dispute this supposition, how close to reality are the
simulated prebiotic experiments?
It is unnecessary for scientists to heat together water, methane,
ammonia and hydrogen (components of the primitive atmosphere),
irradiate the mess with various forms of energy, and then sit back
to wait for a recognizable lifeform to reach its slimy paw over the
edge of the beaker and crawl out onto the lab desktop.
We won’t ever achieve this kind of
completeness, because that takes evolution and the secret to
evolution is time.(But it has been seriously suggested that a
complete artificial seashore be set up to test some of the proposed
mechanisms in the origin of life.)
From chemical equilibria we know the kinds of substances that had to
be floating around in the primitive atmosphere and seas. Protein
molecules ultimately consist of different combinations of only
twenty different amino acids. Nucleic acids are composed of one of
five bases, one of two sugars, and a single type of phosphate group.
As Cyril Ponnamperuma of the
NASA/Ames Exobiology Division once remarked:
"The alphabet of life is extremely
simple; the wide variety of life observed today may be traced to
a mere handful of chemicals."
Abiogenesis research differs markedly
from most other scientific work, in that an unverifiable historical
process is being reconstructed.
It probably is not practical to run
through an entire origin of life "from scratch," so different
criteria must be used to evaluate hypotheses. For instance,
postulates must at least be consistent with known astronomical,
geophysical, and biochemical principles insofar as this is possible.
And stepwise experiments, in which only one step of abiogenesis at a
time is simulated, are reasonable if plausible and appropriate
prebiotic conditions are maintained.
It is believed that the origin of life may have happened very fast,
certainly less than a billion years and possibly less than a hundred
million years. Most estimates today place the creative event in the
primitive seas, roughly 4.2 to 3.6 eons ago.
Prebiotic Synthesis
For many years it was known that mixtures of carbon dioxide, ammonia
and water vapor would produce small amounts of simple organic
chemicals if energy was supplied. But the results of these
experiments were generally very discouraging and the yields
miniscule under these oxidizing conditions. To originate life in
such a poor, thin broth would be well-nigh impossible.
In 1953 a graduate student named Stanley Miller, working
under Nobelist Harold C. Urey at the University of Chicago,
constructed an apparatus to imitate the conditions of the primitive
Earth (Figure 7.1).
Previous investigators had always
assumed the atmosphere to be oxidizing or neutral. Miller and Urey,
following the suggestions of A. I. Oparin in the Soviet Union and J.
B. S. Haldane in Britain during the 1920’s, took the unprecedented
step of devising a reducing environment instead.
Figure 7.1
Miller Apparatus for
Prebiotic Synthesis
In this schematic of the apparatus used
in Stanley Miller's s historical experiment, a variety of organic
compounds are synthesized as the atmosphere of methane (CH4),
ammonia (NH3), hydrogen (H2) and water vapor
(H2O) is subjected to an electric spark discharge.
Circulation is maintained in the system by the boiling water on one
end and the condensing jacket on the ether.
After one week of continuous operation, the water was removed and
tested by paper chromatography. A great abundance of amino acids and
other organics was detected.
Miller mixed together methane, hydrogen, ammonia and water, and
carefully eliminated all oxygen from the system. This gaseous
concoction was then circulated past an electric spark discharge,
followed by a water bath to simulate the primitive sea. After about
one week of continuous operation, the "ocean" had turned a deep
reddish-brown.
The experiment was halted and the contaminated water removed for
analysis. Miller discovered to his amazement and delight that many
amino acids had been produced in surprisingly high yields. Two
percent of the total amount of carbon in the system was converted
into glycine alone. Sugars, urea, and long tarlike polymers too
complex to identify were also present in unusually high
concentrations.
Of course, electrical energy was only one of the many sources of
energy available on the primitive Earth (Figure 7.2).
In fact, ultraviolet radiation was
probably the principle source: UV would have been able to penetrate
to the surface be cause the protective ozone layer in the upper
atmosphere did not yet exist. A Miller-type experiment using
ultraviolet rays and a reducing atmosphere was performed in 1957 by
the German biochemists W. Groth and H. von Weyssenhoff at the
University of Bonn.
Their results closely paralleled those
obtained at the University of Chicago half a decade earlier.
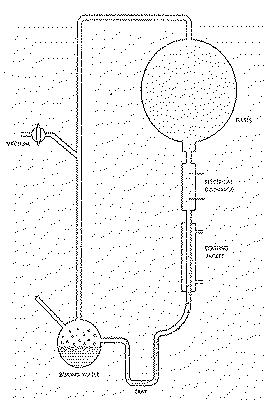
Figure 7.2
Prebiotic Chemical
Evolution on the Primitive Earth
Countless prebiotic simulations have
since been achieved which confirm Miller’s original conclusions.
One bibliography, current through 1974,
lists more than three thousand papers on the subject.1679 An
exhaustive treatment of all of them is clearly beyond the scope of
this book, but the interested reader in encouraged to dive into the
literature (Table 7.1).
Table 7.1 Summary of Prebiotic Synthesis Experiments
through 1975
|
Year
|
Reactants
|
Energy Source
or Catalyst |
Products
|
Ref. #
|
AMINO ACIDS, FATTY ACIDS, AND SIMPLE
ORGANICS |
|
1828
|
Ammonium sulfate, potassium cyanate
|
Thermal reaction
|
Urea
|
1586
|
1913
|
|
Electrical discharge
|
Glycine
|
2257
|
1926
|
CO2, CO, CH4 or H2
|
a-rays
|
Resinous organic material
|
2224
|
1937
|
CO + H2O
|
UV
|
Formaldehyde, (HCO)2 (glyoxal)
|
2317,2266
|
1951
|
CO2, H2O, Fe++
|
a-rays
|
Formaldehyde, formic, succinic acids
|
2226
|
1952
|
formic acid + H2O
|
a-rays (40 MeV)
|
(COOH)2
|
2279
|
1953
|
acetic acid + H2O
|
a-rays
|
Malonic, malic, capric acids
|
2278
|
1953
|
CH4, NH3, H2, H2O
|
Electrical discharge
|
Glycine, alanine, aspartic & glutamic acids
|
2258
|
1954
|
CH2O, H2O, KNO3, ferric
chloride
|
Sunlight
|
Amino acids
|
2261
|
1955
|
Ammonium fumarate
|
Heat
|
Aspartic acid, alanine
|
2267
|
1955
|
CH4, NH3, H2, H2O
|
Electrical discharge
|
Amino acids, hydroxy acids, urea, HCN
|
2259
|
1956
|
CH4, NH3, CO2, H2O
|
|
Amino acids
|
2260
|
1957
|
CH4, NH3, H2, CO2,
H2, CO, N2
|
X-rays
|
Amino acids
|
2262
|
1957
|
CH4, NH3, H2O
|
UV
|
Simple amino acids, fatty acids
|
2269
|
1957
|
Ammonium acetate
|
b-rays
|
Glycine, aspartic acid, diaminosuccinic acid
|
2223
|
1957
|
Glycine
|
Heating w/quartz sand
|
Alanine, aspartic acid
|
2264
|
1957
|
Ammonium carbonate
|
g-rays
|
Glycine
|
2227
|
1959
|
Formaldehyde, hydroxylamine
|
Thermal reaction
|
Glycine, alanine, serine, and others
|
2265
|
1960
|
Hydroxy acids ammonia or urea
|
Heat
|
Glycine, alanine, aspartic & glutamic acids
|
2263
|
1960
|
CO2, H2O
|
g-rays
|
Formic acid
|
2277
|
1961
|
CH4, NH3, H2
|
Accelerated protons
|
Urea, acetone, acetamide |
2316
|
1961
|
NH3, HCN, H2O
|
Heat (70 °C)
|
Amino acids
|
2270
|
1961
|
CO2, C2H4
|
g-rays, or high pressures
|
Long chain fatty acids (C-40)
|
2276
|
1962
|
CH4, NH3, H2
|
b-rays
|
Simple aliphatics incl. amino acids
|
2272
|
1963
|
CH4, NH3, H2, H2O
|
Hypersonic shock wave
|
Many unidentified organic compounds
|
2318
|
1964
|
CH4, NH3, H2O + silica
|
Heat (850 °C)
|
Amino acids
|
2273
|
1964
|
CH4
|
Electrical discharge
|
Higher aromatic hydrocarbons
|
2274
|
1966
|
CH4 + silica contact
|
Heat (1000 °C)
|
Higher aromatic hydrocarbons
|
2271
|
1966
|
Cyanoacetylene, HCN, NH3, H2O
|
Heat (100 °C)
|
Aspartic acid
|
2275
|
1970
|
CH4, C2H6, NH3,
H2O
|
Shock waves ("thunder")
|
Amino acids
|
1664
|
1974
|
CH4, NH3, H2, H2O
|
Electrical discharge
|
Amino acids and simple organics
|
521
|
SIMPLE SUGARS AND CARBOHYDRATES |
|
|
1924
|
Formaldehyde + H2O
|
UV
|
Hexoses, hydroxy acids
|
2280
|
1959
|
Monosaccharides
|
g-rays
|
Polysaccharides
|
2310
|
1962
|
CH2, Ca(OH)2, CH3CHO,
CH2HCHOHCHO
|
Heat (50 °C)
|
2-deoxyribose, 2-deoxyxylose, etc. |
2281
|
1963
|
Formaldehyde + H2O
|
UV
|
Ribose, deoxyribose
|
2243
|
1965
|
Glucose
|
Heat (130 °C)
|
Polyglucose
|
2314
|
1965
|
Formaldehyde
|
UV
|
Ribose, deoxyribose, other sugars
|
2282
|
1965
|
Monosaccharides
|
UV
|
Disaccharides
|
2313
|
POLYPEPTIDES, AMINO ACID POLYMERS, PROTEINOIDS |
|
|
1954
|
Amino acids
|
Heat
|
Amino acid polymer proteinoids
|
2268
|
1954
|
Glycine
|
Electrical discharge
|
Polyglycine
|
2283
|
1959
|
Amino acids
|
X-rays, UV, or g-rays
|
Amino acid polymers
|
2310
|
1959
|
Hot proteinoid material
|
Heat, slow water cooling
|
Proteinoid microspheres
|
2286
|
1960
|
Glycinamide
|
Heat (100 °C)
|
Polyglycine (up to 40-unit strands)
|
2311
|
1961
|
Asperine in water
|
Heat
|
Amino acid polymers
|
2285
|
1961
|
Glycine
|
Heat (140-160 °C)
|
Polyglycine (up to 18-unit strands)
|
2312
|
1964
|
Glycine + Glucose + H2O
|
Heat
|
Amino acid polymers
|
2284
|
1964
|
Amino acids
|
Heat + cold quenching
|
Proteinoid microspheres
|
1702
|
1969
|
Alkanes, Mg++, PO4=
|
UV
|
n-Hexadecane membranes
|
1415
|
1974
|
|
|
Coacervates (a review)
|
1432
|
1974
|
Proteinoid
|
|
Higher bonding in proteinoid microspheres
|
1435
|
1975
|
HCN, H2O
|
|
Heteropolypeptides
|
1438
|
NUCLEIC ACID BASES: PURINES AND PYRIMIDINES |
|
|
1926
|
Malic acid, urea, strong mineral acid
|
Thermal reaction
|
Uracil
|
2292
|
1960
|
Amino acids
|
|
Purines
|
2289
|
1961
|
Malic acid, urea, polyphosphoric acid
|
Heat (100-140 °C)
|
Uracil
|
2244
|
1961
|
Amino acids
|
|
Purines
|
303
|
1962
|
Urea + AICA
|
Heat
|
Guanine, xanthine
|
2281
|
1962
|
HCN, etc.
|
|
Purine intermediates
|
2290
|
1963
|
CH4, NH3, H2O
|
b-rays
|
Adenine
|
2237
|
1963
|
Urea + CH2CHCN or NH2CH2CH2CN
|
Heat (130 °C)
|
Uracil
|
2293
|
1963
|
CH4, NH3, H2
|
b-rays
|
Adenine
|
304
|
1963
|
|
Heat
|
Guanine
|
2238
|
1964
|
Aspartic acid, glutamic acid 16 others
|
Heat (180 °C)
|
Guanine
|
2239
|
1966
|
Urea + AICA
|
Heat
|
Guanine, xanthine
|
2291
|
1971
|
|
|
Thymine
|
2246
|
1971
|
|
|
Adenine, Guanine, Cytosine
|
2241
|
1972
|
|
Zeolite catalyst
|
Purines
|
2247
|
NUCLEOTIDES AND POLYNUCLEOTIDES |
|
|
1962
|
Nucleotides
|
g-rays
|
Polynucleotides
|
2256
|
1963
|
Adenine, ribose, ethyl metaphosphate
|
UV
|
Adenosine triphosphate (ATP)
|
2294
|
1965
|
Nucleosides + phosphates
|
Heat (160 °C)
|
Nucleotides
|
2242
|
1965
|
Bases + metaphosphate ester
|
|
Polynucleotides
|
2235
|
1967
|
Cytidylic acid + polyphosphoric acid
|
|
Cytosine nucleotide
|
2236
|
1967
|
Nucleosides + polyphosphoric acid
|
Heat (22 °C)
|
Nucleotides, nucleoside triphosphates
|
2295
|
1970
|
Imidazole + cyanamide
|
|
Mononucleotides
|
2252
|
1971
|
|
Heat
|
Polynucleotides
|
2251
|
1971
|
Cyanamide
|
|
Mononucleotides
|
2253
|
1971
|
Adenine nucleotide
|
UV
|
Adenine polynucleotide
|
1628
|
1971
|
Cyclonucleoside
|
Heat
|
Polynucleotides
|
1627
|
1971
|
Nucleotide
|
Heat
|
Polynucleotides
|
1626
|
1971
|
|
|
Cytosine nucleotide
|
2254
|
1972
|
Thymine nucleoside
|
|
Thymine nucleotide
|
2245
|
3972
|
Ammonium cyanide + water
|
|
Guanine nucleoside
|
2240
|
1972
|
|
Apatite catalyst
|
Mononucleotides
|
2250
|
1973
|
Cyanamide + AICA
|
|
Mononucleotides
|
2248
|
1973
|
Cyanogen + water
|
Apatite catalyst
|
Mononucleotides
|
2249
|
1974
|
Nucleotides
|
|
Polynucleotides
|
1435
|
1974
|
Nucleosides
|
Heat
|
Oligonucleotides
|
1429
|
1975
|
Nucleoside + ammonium oxalate
|
Heat
|
Nucleotide
|
1439
|
Table 7.2 lists the sources of energy
believed to be present during the first eon or so of Earth’s
history.
Ultraviolet radiation leads the pack.
Carl Sagan and others have completed experiments with UV which seem
to indicate rather high yields for prebiotic amino acids, the
building blocks of proteins. Over the first billion years of
chemical evolution on this world something like a hundred kilograms
of amino acids per square centimeter may have been produced,
resulting in a "soup" of about 1% concentration.
This is the approximate consistency of
chicken bouillon.
Table 7.2 Energy Available for Synthesis of Organic
Compounds on the Primitive Earth
|
Source of Energy
|
Energy Available
(joules/meter2/year)
|
Solar radiation, all wavelengths |
1.1 × 1010
|
Ultraviolet, |
l < 3000 Ang |
1.4 × 108
|
|
l < 2500 Ang |
2.4 × 107
|
|
l < 2000 Ang |
3.6 × 106
|
|
l < 1500 Ang |
1.5 × 105
|
Electrical discharges |
1.7 × 105
|
Decay of crustal K-40, 4 eons ago |
1.2 × 105
|
(Decay of crustal K-40, today) |
(3.4 × 104)
|
Shock waves |
4.6 × 104
|
Heat from volcanoes |
5.4 × 103
|
Meteoritic impact |
4.2 × 103
|
Cosmic rays |
6.3 × 101
|
But ultraviolet radiation is a two-edged
sword.
While it may be the most abundant form
of energy for molecule building, it is also the most destructive.
Early researchers were concerned that organics would be destroyed as
fast as they were created. Fortunately, the primitive oceans
probably turned opaque like the brownish glop in Miller’s apparatus
rather quickly.
Vital chemicals newly synthesized and
carried a short distance beneath the surface of the soup by
convection undoubtedly escaped decomposition.
Of the remaining energy sources, electrical discharge was the most
potent. As much as 5-15% of the carbon in a mixture of methane,
ammonia and water may be converted to amino acids and other organics
by the energy of the discharge. Various forms of ionizing radiation
give high yields as well. a particles, b particles, and g rays were
common on the surface of the primitive Earth because of the presence
of intense natural radioactive sources in the crust - such as
potassium-40, thorium-232, and isotopes of uranium.
Volcanic heat was another prebiotic power supply. It has been shown
that lava-heated seawater and underwater volcanoes may be effective
in producing biologically important compounds. Heat and sonic energy
would have been released by infalling meteorites - certainly a
significant factor in the environment of the primitive solar system.
In fact, experiments performed recently
by Bar-Nun and others have conclusively demonstrated that as much as
30% of the nitrogen in an ammonia atmosphere can be converted into
amino acids in this manner. Torrential rains have even been
suggested as a possible source of energy for prebiotic synthesis,
and experiments have shown that a flask of formaldehyde, allowed to
stand for a few days at room temperature, will produce some simple
sugars.
The great lesson appears to be that the exact nature of the power
supply is relatively unimportant.
Amino acids, sugars, and other chemical
precursors to life probably arise on any planet possessing an
initially reducing atmosphere and quantities of hydrogen, carbon,
nitrogen and oxygen in gaseous reduced form - regardless of the
particular source, or sources, of energy available.*
* Other factors may also
be important. For instance, early-type stars (F) are more likely to
emit ultraviolet radiation in copious quantities than are late-type
stars (K, M). The speed of chemical evolution in primitive planetary
environments may actually slow as we move from class F through
classes G to K stars among habitable solar systems.
Proteins and
Cells
The cell is the fundamental biological unit and a common denominator
among all terrestrial lifeforms.
Living things on this planet are
made up of cells which vary in size from less than one micron to
several centimeters in diameter. While the simplest organisms are
unicellular, the typical human is an ambulatory assemblage of from
fifty to a hundred trillion (1014) individual cells.
The cellular construction of Earth life is remarkably uniform:
Similar water content, similar kinds of proteins, similar lipids and
so forth. All have at least one membrane, perhaps no more than 100
Angstroms thick, which protects the inner workings from the harsh
vagaries of the external environment. The first protobionts
undoubtedly had no such complex organizational qualities. But how
can structure arise in the first place?
It has been shown by Ilya Prigogine that thermodynamic chemical
systems may develop certain states wherein some of the chemical
constituents have periodic, oscillating values. A biologist, J.
Pringle, has demonstrated that initially homogeneous systems can
undergo a progressive change, leading to the appearance of "spatial
heterogeneity."
That is, structure can arise
spontaneously. These two treatments of the problem of organization
suggest that mechanisms may exist for collecting material into
small, localized concentrations, perhaps leading to ordered
structures we would recognize as cells.
But to build cells, we must have protein. Protein is the most
fundamental construction material, used in building cell walls,
enzymes, and so forth. To make proteins, there are two requirements.
First, we need an abundance of amino acids. From our discussion
above, we see that this is virtually inevitable on any normal world
possessing at least small aqueous oceans and a primitive hydrogenous
atmosphere.
Second, there must be some way to hook up a long string of amino
acids into a polymer of protein. Polymerization (linking together)
of amino acids leads to the production of protein, which can then be
used for cell-building.*
* We will not discuss here the
significance of molecular optical activity. The curious reader is
referred to Sagan, Jackson and Moore, Glasstone, Gabel and
Ponnamperuma, Miller and Orgel, Ulbricht, Hochstim, Bonner et al,
Wald, and Walker.
It is true that even dilute primordial
soups can coagulate into gelatinous masses. But such conditions are
far from ideal. In all likelihood, most prebiotic syntheses probably
took place in local regions of increased concentration. The
efficient construction of amino acid polymers undoubtedly occurred
elsewhere than in the open seas.
Numerous concentration mechanisms have been proposed which might
conceivably lead to the creation of small pockets of more potent
broth. The simplest method is evaporation. Primordial soup, caught
in a narrow, shallow lagoon, would slowly thicken as the water that
held the components in solution evaporated away. As suggested by
Miller and Orgel, similar effects result from slowly freezing the
solution in the lagoon: The solvent freezes out in the pure form
first, leaving the solute concentrated in ever-smaller quantities of
solvent.
A combination of air-water and water-solid interfaces provides
mechanical consolidation of suspended matter, as evidenced by the
accumulation of scums and oil slicks near coastlines. Another
possibility is that organic compounds may have been trapped on solid
surfaces such as aluminum silicate clays, quartz, and other minerals
which allow polymerization reactions to proceed.
To date, however, there is really only one proven method which
yields polymers of amino acids under plausible prebiotic conditions.
Dr. Sidney W. Fox at the University of Miami has obtained long-chain
molecules with the following essential properties:
-
They contain all amino acids
common in contemporary terrestrial organisms
-
They have high molecular weights
(the chains are relatively long)
-
They are "active" because they
interact in the catalytic or rate-enhancing sense. (This
anticipates metabolic activities mediated by enzymes - which
are also proteins
-
They are as heterogeneous as
contemporary proteins
-
They yield "organized units"
upon contact with water which have many properties in common
with modern cells.
Dr. Fox calls his substances "proteinoids,"
because they greatly resemble living protein polymers.
His method for producing them is quite
simple. A mixture of amino acids is cooked at 120-170 °C for a few
hours, and substantial yields (10-40%) of polymeric material are
obtained.
Fox decided to test his method under more realistic field
conditions. He secured a large piece of lava from the site of an
Hawaiian volcano. The temperature of the rock was raised to 170°C,
and the appropriate amino acids seated in a small depression at the
top. Heating continued for several hours, after which the lava was
washed off with a small spray of sterilized boiling salty water - as
might have occurred naturally near a volcanic shoreline in ancient
times.
Proteinoid polymers were formed, but
there was more! To Dr. Fox’s surprise, billions of tiny "microspheres"
appeared in the wash water: spherical, microscopic particles of
uniform diameter bearing a striking resemblance to living cells
(Figure 7.3).
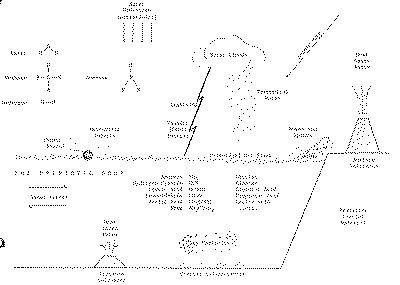
Figure 7.3
Possible Model
Protobionts
Thermal Proteinoid Microspheres
|
Structured thermal proteinoid
microspheres.
|
These proteinoid microspheres
were produced by slowly cooling a hot, clear solution of thermally
polymerized amino acids.
|
|
(At right) Various stages of binary
fission of proteinoid microsphere "protocells"
|
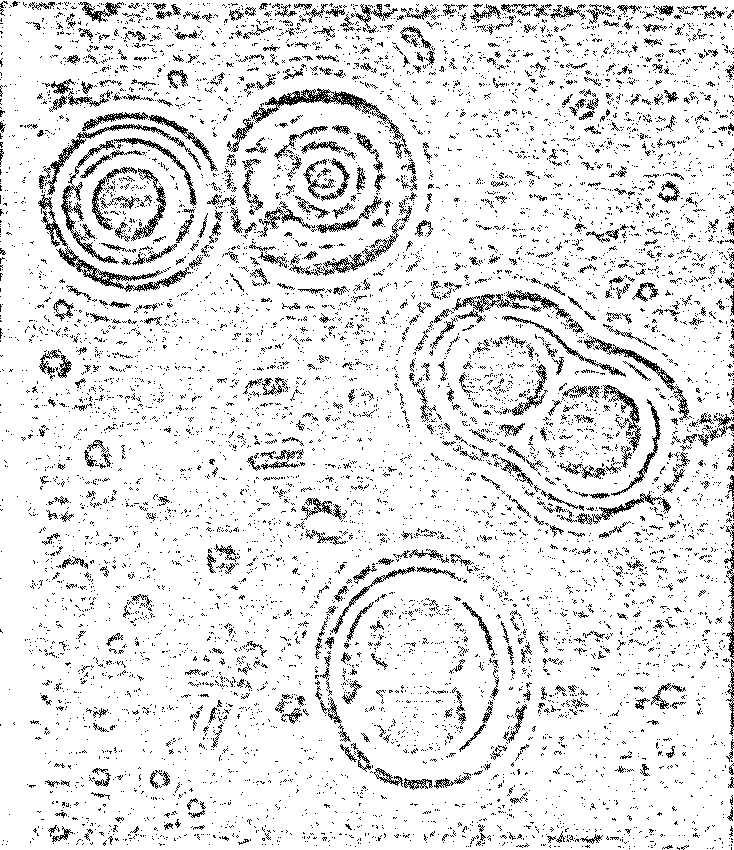 |
|
(Above) Parent microspheres spout
buds.
|
|
(At right) Second-generation
laid on second generation microsphere. After the bud grows to maturity,
it sprouts its own new buds. Is this a form of incipient reproductive
capability?
|
|
The Miami scientist presented a scenario
for the origin of microsphere protocells in prebiotic times:
-
hot lava meets soup
-
water boils away, leaving sticky
brown goop on lava
-
contact with water (rain, sea
spray, etc.) causes proteinoids to assemble themselves
-
microspheres are washed back
into the soup
These initial experiments were completed
nearly two decades ago, and since that time Fox and his colleagues
have refined their methods and perfected their theories on the
origin of cells and life.
Protein-like materials are now produced
with molecular weights ranging from 3000 to 10,000 under plausible
primitive Earth conditions. And it has been shown that a primitive
“cell” with most of the attributes of life can arise spontaneously
in a very brief period of time.
Detailed studies of microspheres have confirmed the researchers’
initial optimism. What makes these spherules so unique is their
“active” nature.
Dr. Fox has observed and recorded the
following characteristic behavior of his proteinoid microspheres
under various chemical and physical conditions:
-
Spherical shape - 0.5 to 7.0
microns, uniformly
-
Single-walled membranes (like
plants) and double-walled membranes (like animals)
-
Simulation of osmosis -
microspheres swell and shrink in response to changes in the
chemical environment
-
Selectivity of diffusion -
microspheres possess semipermeable membranes analogous to
those of living cells. For instance, in one case Fox
discovered that polysaccharides were selectively retained
under conditions in which monosaccharides diffused freely
through the microsphere walls. (Polynucleotides and other
organics are also absorbed from aqueous solution)
-
Cleavage - a kind of binary
fission of a single “cell” has been observed in acidic
proteinoid microspheres
-
Motility - the microspheres,
when viewed under a microscope, move non-randomly in
preferred directions under certain special conditions. The
addition of ATP appears to enhance the movement
-
Budding - buds appear
spontaneously on proteinoid microspheres allowed to stand
undisturbed in their mother liquor
-
Growth by accretion - buds which
have been liberated by mild heating or electric shock will
swell by diffusion to the same approximate size as the
“parent” cell
-
Proliferation through budding -
second generation budding has frequently been observed on
buds that grew to the size of normal microspheres. The buds
are apparently engaging in a kind of “reproduction”
-
Formation of junctions -
microspheres are capable of approaching one another and
physically attaching together in a more or less permanent
fashion
-
Transmission of information -
when two spheres have joined, small proteinoid
microparticles within the larger sphere are observed to pass
through the junctions. The whole process is highly
suggestive of microbial conjugation
-
Stability - the activity of the
proteinoids does not diminish with storage over a period of
5-10 years.
The best-known of all physical cell
models prior to the discovery of proteinoids was the coacervates,
thoroughly researched by the Soviet biochemist A. I. Oparin, the
Dutch biochemist H. G. B. de Jong, and others.
Coacervates are produced by combining
solutions of oppositely charged colloids such as gelatin or histone
with gum arabic. When solutions of the two substances are
commingled, they interact to yield clusters of microscopic
structures having the appearance of tiny liquid droplets.
Coacervates have many interesting properties from the point of view
of the origin of life.
For instance, after these uniform spherules have aggregated, they
are able to absorb various simple organic molecules from the
external medium (sugars, dyes, etc.). However, Oparin has admitted
that this process quickly leads to static equilibrium, and the
coacervate "protocell" then becomes a passive system, unstable and
prone to break-up upon standing.
Another property of coacervates is their
ability to convert certain chemical monomers to polymers after
diffusion through the "cell" wall, although it is generally
recognized that the dynamic behavior of these droplets is fairly
limited.
There is another reason why coacervates,sulphobes,"biphasic
vesicles," and many other prospective pseudocells do not compare
favorably with Dr. Sidney Fox’s microspheres as model protocells.
Coacervate droplets are formed from
polymers which themselves were synthesized by living organisms. The
gum arabic used to manufacture Oparin’s droplets was not produced
abiogenetically, nor is it at all clear how this might be done. The
great advantage of the microspheres is that they are the direct
product of single, simple amino acids - amino acids that must have
been common on the shores and seas of the primitive Earth eons ago.
Of course, no biologist today would claim that proteinoid
microspheres are alive in the sense of representing the first
protocell.
And yet, to the extent that they
self-organize, accumulate information from their surroundings, and
exhibit both structure and behavior, they are certainly near the
borderline of life.
Nucleic Acids
and DNA
In the previous section it was mentioned that there are two
requirements for the production of proteins. First, there must be
amino acids, and second, there must be a way to hook them together
to form polymers.
There is, however, a third requirement for the origin of living
systems on Earth. It will be recalled from the discussion of the
definition of life that "it is the business of life to accumulate
information and complexity."
Let us consider this mandate in view of
the problem of building proteins.
To abiogenetically produce a living system, that system must be
capable of accumulating information and order from its environment.
The proteins constructed by a cell must have the proper architecture
for whatever job needs to be done. So our third requirement may be
stated: There must be a way to hook the amino acids together in the
correct sequence. Any old proteins will not do - they must be the
right ones.
There exist simple chemical techniques to achieve this kind of
ordering. One common example is called "autocatalysis" by chemists.
Autocatalysis is a way for a process to catalyze its own production.
Once a tiny bit of it has been produced, that bit catalyses the rate
of reaction to yield still more, and faster.
Aside from this simple selective feedback effect, the development of
molecular self-replication was probably the most critical single
event in the origin of life on Earth. The origin of replication and
the genetic code, as opposed to the origin of proteins and cells,
allowed natural selection to begin to operate on stored information.
And once evolution begins, selective advantages of superior
membranes and of multicellular colonies can be expressed in the form
of increased organismal complexity.
DNA - the primary information-carrying molecule used by all
lifeforms on this planet - is a polymeric nucleic acid (Figure 7.4).
We’ve already seen how easy it is to get amino acids and their
polymers.
But what about nucleic acids?
Can they
be demonstrated in prebiotic synthesis experiments, along with their
polymers?
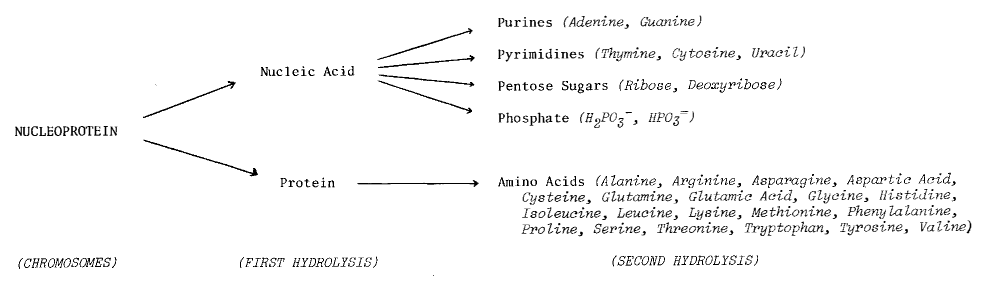
Figure 7.4
The Role of Nucleic
Acids in Terrestrial Biochemistry
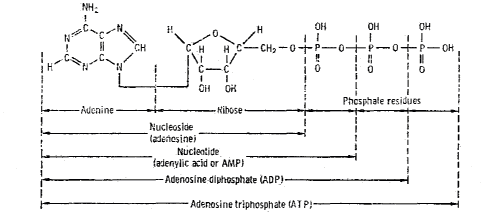
Chemical Structure of Nucleic Acid (from Glasstone72)
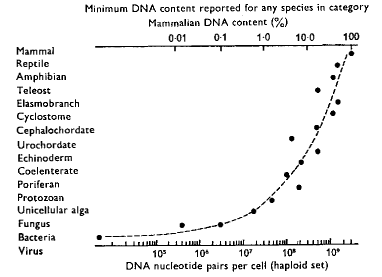
DNA Content (figure from Britten and Davidson2568, in Kohne1654)
In 1963, Dr. Cyril Ponnamperuma
managed to synthesize adenine (one of the two most important nucleic
acid purine bases) under simulated primitive Earth conditions.
The NASA scientist and his three
colleagues used a Miller-type apparatus, and began their synthesis
with nothing more than methane, ammonia and water in the system. The
mixture was bombarded with energetic electrons, and about 0.01% of
the carbon in the methane was converted into adenine.
This is highly
significant because adenine is useful, not only for making DNA, but
also RNA, ATP, ADP, FAD, and a host of other critical
life-molecules.
In a related experiment two years later, Dr. John Oró of the
University of Houston and A. P. Kimball produced adenine is a closed
reaction system which included ammonia, water, and hydrogen cyanide.
Heat was supplied as the energy source, and this time the production
of the purine base rose to 0.5% of the available carbon.
This value
was observed over a wide range of chemical conditions, indicating
the relative ease with which this complex molecule must arise in a
plausible prebiotic environment. The synthesis of the other
important purine, guanine, has also been convincingly demonstrated.
There have been various attempts to fabricate the three major
varieties of pyrimidine bases which are also necessary in the
production of nucleic acids. However, the appearance of these
substances under conditions similar to the primitive Earth has not
been investigated as thoroughly as the purines.
One experiment that yields a hefty 20% of cytosine requires a
three-step process involving methane and nitrogen initially to
create a cyanoacetylene intermediate, which then goes on to produce
the pyrimidine when combined with cyanate ion. Uracil, another
pyrimidine, is obtained in very good yield by the direct hydrolysis
of cytosine - a prebiotically reasonable reaction.
All the pyrimidines have been
synthesized in environments at least arguably analogous to that of
the early Earth.
Prebiotic assembly of purines and pyrimidines into full-fledged
nucleotides has proven more difficult, and intensive investigations
are now underway to determine and eliminate the problem. The main
obstacle to success seems to be the formidable complexity of the
nucleotide molecules themselves. While bases and sugars are
relatively easy to produce, combining them together is a much harder
task.
Nevertheless, demonstrations of nucleotide synthesis under
geologically plausible constraints have been made. One such
technique involves the use of a mediating mineral called apatite,
which contains phosphates and oxalate ion, in an "evaporating pond"
scenario.
We are not quite home yet. Just as amino acids needed polymerization
to become protein, so must nucleotides by polymerized into DNA. What
progress has been made in the prebiotic synthesis of polynucleotides?
The experimental record is admittedly spotty. When adenine
nucleotides were heated in the presence of polyphosphate for 18
hours at 55 °C, adenine polynucleotide polymers were obtained
ranging from 20-30 nucleotides per chain. However, in the words of
the experimenter,
"the concentration of the reactants
had to be as high as possible when the formation of high
polymeric material was desired."
That is, unless quite artificial
conditions were contrived, the adenine nucleotides could not be
forged into very long chains. In another experiment, solutions of
adenine nucleotide were irradiated with UV light. Long chains were
again obtained, but only when extraordinarily high concentrations of
polyphosphate were maintained.1628 Under similarly unrealistic
conditions, uracil polynucleotides with chain lengths ranging from
10-50 units have been found.
One good experiment has been performed by John Oró and E.
Stephen-Sherwood, using a plausible "evaporating lakebed"
scenario and temperatures from 60-80°C. Uracil two-unit chains were
formed with a yield of 23%, and three-unit segments with a 12%
yield.
Cytosine polynucleotide chains were
obtained by these experimenters with up to six nucleotides in
straight-line linkages. Thymine polynucleotides 2-12 units long were
produced when an unreasonable chemical environment was used; with
more closely matched prebiotic conditions, five-unit chains were
obtained in yields of 1% or less.
The polymerization of some nucleotides has proven unexpectedly
difficult, partly because of the inevitable formation of unnatural
side chains and partly because the reaction just doesn’t seem to
want to go. Various solutions to these problems have been suggested.
For instance, there are enzymes - ordinary proteins - that are
capable of catalyzing these polymerization reactions with ease.
These enzymes, or enzymes like them,
could have arisen by nonbiological means. If this is the case,
claims one researcher,
"such catalysts may have been
responsible for the first polymerization of nucleotides on the
primitive Earth."
So at present, here is where we stand.
Purines and pyrimidines are
comparatively simple to manufacture abiogenetically. The assembly of
nucleotides has also met with some limited success, but to date it
has proven difficult to synthesize more than six-unit polymeric
chains in a prebiotically plausible way.
Can these short strands alone make a stab at primitive replication?
Dr. Leslie Orgel at the Salk Institute in San Diego, California,
mixed up a solution of nucleic acids that might be considered
prebiotically reasonable. He then placed some of the six-nucleotide
polymers in his specially-enriched "soup."
The short-chain DNA polymers correctly
replicated themselves once out of every ten tries.
Early Biological
Systems
Thus far we have concentrated on the parallel development of
polymeric amino acids (proteins) and polymeric nucleotides (DNA).
We’ve seen that Dr. Sidney Fox’s
proteinoid microspheres exhibit many properties which are strikingly
similar to those displayed by contemporary living cells. We’ve also
seen that Dr. Leslie Orgel has succeeded in demonstrating accurate,
if erratic, replication in primitive polynucleotides. And yet,
despite these remarkable achievements, the great final question
remains untackled: How and when did the first living organism arise?
*
* There are countless side
issues that cry out to be discussed at this point, but which
unfortunately can be given only a passing nod. First of all, there
is the absolutely fascinating question of the genetic code. As is
well-known, genetic information is written on the DNA strand in
short, three-nucleotide "words" called codons. By properly reading
these encoded blueprints, a cell can construct exactly the right
protein molecules.
The answer is as unsatisfying as it is precise: No one knows.
The arguments on this score smack of the
"chicken-or-the-egg" controversy. It is unknown at present if
proteins and protocells came first, to be followed later by
replicative nucleic acids, or whether the nucleic acids were first,
and from them the cells later spawned.
It has been fairly clearly demonstrated that life as we know it
could not have arisen if either one or the other was wholly absent.
Organisms lacking nucleic acids would have no means of achieving
genetic continuity and evolutionary progression, while organisms
without proteins would find themselves severely limited in their
ability to utilize the chemicals in their environment. Some manner
of coevolution seems to be indicated.
One theory holds that nucleic acids evolved some kind of boundary
layer, a proteinous skin to protect themselves from their
surroundings - the so-called "naked gene" theory. When this
invention inhibited or prevented reproduction, the parent nucleic
acid molecule became extinct. When the new boundary layer served to
protect the DNA without interfering with replication, these were the
"protobionts" which survived.
There is some experimental evidence to support the view that
polynucleotides might be able to influence protein synthesis
directly.
To do this, they must cause a selective
linear organization of amino acids, and must facilitate amino acid
polymerization. Unfortunately, other studies have shown that the
interaction between polynucleotides with individual (monomeric)
amino acids is relatively weak.
More convincing, perhaps, is the idea that cells were first.
Self-assembly in molecular structures has been known for many
decades, and experimental evidence to date favors the easy synthesis
of proteins in comparison to polynucleotides.
Sidney Fox has remarked that the
sequence:
protoprotein —> protocell —>
nucleic-acid-coded contemporary cell,
...is the most valid evolutionary
sequence because it proceeds from the simple to the more complex.
The primitive protocell, as modeled by the proteinoid microspheres,
could have exhibited many of the properties customarily regarded as
belonging only to "living" things. Under Fox’s theory, the cell
would have developed nucleic acids to serve its ends, rather than
the other way around.
One final piece of evidence seems to argue for the primacy of cells.
In 1974, Dr. Fox and his colleagues published some experimental
findings on micro-spheres which seem to imply that the proteinoid
protocell can do everything optimistically predicted for it.
The abstract of the paper reads, in
part:
Proteinoid microspheres of
appropriate sorts promote the conversion of ATP to adenine
dinucleotide and adenine trinucleotide. When viewed in a context
with the origin and properties of proteinoid microspheres, these
results model the origin from a protocell of a more contemporary
type of cell able to synthesize its own polyamino acids and
polynucleotides.
We’ve seen that scientists have
discovered a relatively smooth chain of synthesis from the stuff of
stars to the stuff of life.
On the basis of pre biotic experiments
performed to date, it is probable that most of the organic molecules
of life with a molecular weight less than 1000 spontaneously
appeared in significant quantities during the early years of our
world. While a number of problems remain, most indications are that
the origin and development of life on Earth had a certain
inevitability about it.
From the simplest compounds present when our planet first congealed
about 4.6 eons ago, to the first viable protobiont some half a
billion years later, the patterns of development and the upward
march of complexity seem unavoidable. Only the most general
conditions must be needed for carbon-based life to arise: A body of
water, a primitive reducing atmosphere, some source of energy, and
lots of time. Life, in Soviet Academician Oparin’s own words, is "an
obligatory result of the general growth of the universe."
Even now we humans just begin to suspect the truth: The universe is
not ours alone to keep.
For instance, a series of three adenine nucleotides in a codon tells
the cellular machinery to use one molecule of an amino acid called
lysine at that location. Three guanines in a row means that a
molecule of the amino acid glycine should be used. One by one, the
codons tell which amino acid to use and in what order, and proteins
are built up in precisely the right way.
What is the origin of this marvelous code?
Is a three-nucleotide codon somehow
optimal, or would four have been more evolutionarily efficient? Why
not the simplicity of only two? And what determined the rules of the
coding itself? Three guanines mean glycine to a virus, a dandelion,
or a human. Is the code somehow efficiency-maximized or
error-minimized? (It appears to be!)
What is the origin of chromosomes, and the true purpose of genes?
These are important questions for xenobiologists to be asking,
because the universality of our genetic mechanisms will determine
the limits of variation that can be expected in alien
biochemistries.
For xenologists, of course, there are
far more fundamental issues that must be raised. For example, why
must genetic information be stored digitally in a linear sequence of
monomer units? Could not some form of analog system serve? What of
the possibility of genetic systems whose information was stored,
replicated and transcribed in a planar fashion rather than linearly?
Dr. Francis Crick has pointed out that on Earth, DNA is used for
"replication" and proteins are used solely for "expression" or
"action."
Is it possible, he asks,
"to devise a system in which one
molecule does both jobs, or are there perhaps strong arguments,
from systems analysis, which might suggest that to divide the
job into two gives a great advantage?"
Others have echoed this idea.
Similarly, Michael Arbib of the University of Massachusetts at
Amherst questions,
"whether it is necessary for any
lifeform to have a genotype distinct from a phenotype; in other
words, whether we have to have a program to direct growth and
change, or whether in fact the organism might be able to
reproduce itself as a whole."
Crick seems to agree, suggesting that it
might be possible to "design a system which was based on the
inheritance of acquired characteristics." (At least one science
fiction story has been written along these lines)
Arbib also wonders:
"One might imagine some planet whose
beings reproduce by xerography with no gene required!"
The possibility of inheritance without
genes has been suggested before, although in a different context. (A
general review of replication was published in 2004 by Freitas and
Merkle.)
And we must take care not to be guilty of "nucleic acid chauvinism."
We are familiar with only one molecular replicating system, but
there is no reason why others should not be possible.
Gordon Allen writes:
"Life on other planets need not be
based on nucleic acids or proteins if their catalytic functions
can be otherwise provided."
Dr. Alexander Rich at MIT also
suspects that the functions of Earthly nucleic acid are not unique.
Rich believes that,
"other molecules could be used to
form other polymers which could be used as information carriers
for living systems."
Later, he elaborates:
I think it would be amusing to make
a chemical system of complementary polymers based on monomers
that are not nucleic acid derivatives, simply to demonstrate
that it can be done. In about ten years’ time, I think we will
have a well-developed field of synthetic polymeric information
carriers that will give us a great deal of insight into our own
terrestrial system.
That another system is possible
might have relevance, if not to biology on this planet then
perhaps to another.
Clearly, a search should be made for
non-nucleic acid self-replicating molecules. Exotic systems based on
silicon, boron, or nitrogen-phosphorus chemistries are possible:
Specialists in these fields expect an abundance of compounds
comparable to that of carbon chemistry.
But we must not anticipate the subject
matter of the next chapter.
|